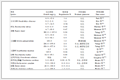

The new challenges for the development of NH3-SCR catalysts under new situation of energy transition in power generation industry
English
The new challenges for the development of NH3-SCR catalysts under new situation of energy transition in power generation industry
-
Key words:
- NH3-SCR
- / NOx reduction
- / Power generation
- / Ultra-low temperature
- / Wide temperature windows
-
1. Introduction
Nitrogen oxides (NOx), as one of the main air pollutants, could cause some serious environmental problems such as acid rain, photochemical smog [1–3]. Furthermore, some researchers found that the nitrogen oxides could also damage people's lungs and contribute to severe lung disease in recent years [4–6]. Therefore, how to reduce the concentration of NOx in the air is important for the treatment of air pollution. The industrial production was one of the main sources of NOx in the air. According to the statistics [7–9], the industrial NOx mainly comes from the flue gas of some industrial sectors which use fossil fuels and nitric acids (e.g., coal-fired power plants, municipal waste incineration, cement plants, steel plants). Therefore, in most countries of the world, the emission standard of NOx in these industrial sectors was very strict (e.g., coal-fired power plants: USA: 135 mg/m3, EU: 200 mg/m3, China: 100 mg/m3) [10]. In order to meet the increasingly stringent emission standards of industrial NOx, a lot of technologies have been applied to reduce the concentration of NOx in flue gas from the industrial production. The general strategies for controlling NOx emissions include selective non-catalytic reduction (SNCR), selective catalytic reaction (SCR), wet scrubbing, adsorption, electrochemical method and so on [11–16]. Among them, NH3-SCR is becoming the most widely applied technology of NOx removal in some industrial sectors due to its high efficiency and simplified operational procedure [17–20]. Generally, the main deNOx reactions in NH3-SCR are shown below (Eqs. 1–5) [21–23]:
(1) (2) (3) (4) (5) The general definition about NH3-SCR reactions is "Standard SCR" or "Fast SCR". And the fast SCR is completed more quickly than standard SCR when the molar ratio of NO/NO2 is 1:1 [24].
For NH3-SCR technology, the development of low-cost and high-performance SCR catalysts is the key to achieve highly efficient removal of NOx. In the past few decades, V2O5–WO3/TiO2 catalysts have been widely used in industrial deNOx on account of its high efficiency in the temperature window of 300–400 ℃ [25–30]. However, with the expanding of the application scenarios of NH3-SCR, the traditional V2O5–WO3/TiO2 catalysts are unable to meet the need of NH3-SCR under different working conditions in different industries. As shown in Table 1, we summarized and compared the main characteristics of the flue gas in several typical industries.
Table 1
According to this, the central challenges for the development of NH3-SCR catalysts in different industries were proposed. And compared with other industries, the NH3-SCR technology is facing some new challenges which are deserved to be discussed carefully in energy generation industry due to the deep transformations of energy sector. Specifically, with the prosperous development of new energy generation sector, the technology of electricity generation is going through a transition from traditional coal-fired to new energy power generation [31]. And the traditional power plants would account for decreasing percentage of the total electricity generating capacity in the near future. The coal-fired power plants would operate under a low-load condition for a long time. Therefore, the temperature of flue gas exhausted from coal-fired boiler would drop to about 200 ℃. The activity of V2O5–WO3/TiO2 rapidly declines when the working temperature is below 300 ℃ or above 400 ℃. Thus, the catalytic activity of V2O5–WO3/TiO2 can not meet the demand of deNOx efficiency with the temperature below 300 ℃. Therefore, how to develop novel SCR catalysts which could maintain high deNOx efficiency in a wide temperature window from 200 ℃ to 400 ℃ is an urgent demand for the further application of NH3-SCR technology in coal-fired power industry.
In addition, SO2 poisoning for NH3-SCR catalysts is another well-explored issue [32–35]. And it was generally accepted that the poisoning mechanism was the deposition of NH4HSO4 (ABS) and the sulfation of metal active sites [36]. As is reported, the deposition amount of ABS on catalyst surface would dramatically increase when the reaction temperature dropped to below 300 ℃ [32,37]. Therefore, another challenge for the development of NH3-SCR catalysts in coal-fired power industry was how to improve SO2 resistance of NH3-SCR catalysts under low working temperature.
On the other hand, on account of the non-renewability of coals, people are trying to use renewable biomass energy to replace it as the main fuel to generate electricity. Thus, the technology of NH3-SCR is facing an entirely different condition in new energy generation industry. In recent years, people come to recognize that municipal wastes could be used as a renewable biomass fuel [38–43]. Municipal waste incineration power is becoming an important new energy generation technology. The temperature of flue gas generated during municipal waste incineration was generally at 150–180 ℃. Therefore, developing NH3-SCR catalyst which could work at ultra-low operation temperature (≤ 200 ℃) is highly demanded for municipal waste incineration. In addition, the flue gas of municipal waste incineration includes lots of water vapor (≥ 25%) and SO2. Improving the H2O + SO2 resistance of NH3-SCR catalysts was another challenge for deNOx in municipal waste incineration industry.
The development of NH3-SCR catalysts has received increasing attention in the past several decades [44,45]. As shown in Fig. 1, the number of publications on the topic of "SCR catalyst" increased dramatically in the past few decades. However, no reviews summarize the challenges of NH3-SCR catalysts during the energy transition of power generation industry.
Figure 1
In this work, we detailly analyze the development of NH3-SCR catalysts in electric industries (coal-fired power plants and municipal waste incineration). And subsequently, we further discuss that the general strategies on solving these problems for the different types of catalysts by giving some specific examples. At last, we also propose the future research challenges and directions of deNOx in electric industries.
2. SCR catalysts in coal-fired power plants
2.1 Broad temperature window (200–400 ℃)
NH3-SCR technology has become one of the most efficient ways to remove NOx in coal-fired power plants [46]. However, in order to combat climate change, the electric systems need turn to a low-carbon and sustainable development mode in the near future. The new energy generation plants are gradually replacing traditional power plants. Therefore, the traditional coal-fired power boilers would have to operate under a low load condition. The temperature of flue gas generated in coal-fired power boiler would decrease to about 250 ℃ under a low-load condition. However, traditional V2O5-TiO2 only could maintain a high catalytic activity in a narrow temperature window (300–400 ℃). And it could not meet the demand of future coal-fired power plants. The development of new catalysts and modification of traditional V2O5-based catalysts which can work in a broad temperature window has been a pressing demand in coal-fired power industry.
As shown in Fig. 2, in general, the mechanism of standard NH3-SCR reaction includes acid and redox circle. In terms of L-H mechanism, the NH3 is absorbed on acid sites to generate NH4+, and the active nitrates generated by the oxidation of NO further react with NH4+, leading to the generation of NH4NO2/NH4NO3 species which degrade to N2 and H2O. In terms of E-R mechanism, the NH3 is firstly absorbed on acid sites and dehydrogenated to -NH2 while the high valence metal is reduced. Subsequently, the -NH2 reacts with NO to form -NH2NO which decomposes to N2 and H2O to complete the acid circle. The low valence metal is oxidized to high valence by O2 and further to generate H2O to complete the redox circle. As is reported [47], the acid and redox circle would significantly slow down with the decrease of reaction temperature. Therefore, how to build up the acid strength of active metal sites and improve their redox properties are the key to improve the catalyst activity of NH3-SCR catalysts.
Figure 2
The development of catalysts generally focused on traditional V-based oxides, novel Ce/Fe-based oxides and ion-exchanged zeolites. And the general strategies were the surface modification, structural designment of catalyst and so on. According to our statistic in Table 2, most catalysts have achieved high NOx removal efficiency in a broad-temperature window from 200 ℃ to 450 ℃ [48–69].
Table 2
Table 2. The summary about the literature data for widen operating temperature windows on NH3−SCR reaction.2.1.1 The modification of V-based catalysts
The traditional V2O5/TiO2 catalyst work within a narrow temperature range between 300 ℃ and 400 ℃, nevertheless, its operating temperature could be broadened by modifying catalyst surface or using catalyst support. Zhao et al. [50] reported a S-N doped V2O5/TiO2 catalyst and its NOx removal efficiency achieved nearly 100% within a temperature range of 240–450 ℃. The NH3-TPD and H2-TPR curves indicated that the introduction of S-N built up the acid strength of V2O5/TiO2 and enhanced the redox property of V2O5/TiO2 catalyst. In their another publication, Ti3+ were introduced to V2O5/TiO2 catalyst to improve its low-temperature catalysis performance [70]. Furthermore, Cao et al. [71] found that the co-doping of Ce4+ and Zr4+ could improve the denitration performance of V2O5-WO3/TiO2.
The broad temperature performance of V2O5-based catalysts could also be improved by modification of catalyst support. The modification of catalyst support can be classified into two groups: the modification of traditional TiO2 support and the introduction of novel catalyst support.
Lian et al. [25] pretreated TiO2 support at 850 ℃ and deposited vanadium species on its surface. They found that the NH3-SCR performance of catalyst using pretreated TiO2 as the support could achieve nearly 100% deNOx efficiency at 240 ℃ and above. Si et al. [72] successfully increased the deNOx efficiency of V2O5/TiO2 under the working temperature of 250–350 ℃ by doping Sn into rutile TiO2. Song et al. [73] successfully synthesized microporous TiO2 as the support of V2O5 catalyst which enhanced the NOx conversion to nearly 90% at the working temperature of 250 ℃ below.
For the design of novel catalyst support, Liu et al. [48] prepared ZrO2 supports with diverse morphologies as the supports of V2O5 by self-assembly methods. And they found that the interaction between mesoporous ZrO2 support and surficial vanadium species is beneficial for the increased proportion of low valence vanadium and absorbed oxygen. And the catalyst activity test indicated that the NO conversion over V/MZ (V2O5 deposited on mesoporous ZrO2) achieved nearly 95% between 225 ℃ and 425 ℃.
2.1.2 The development of Fe-based catalysts
The Fe-based metal oxides as a promising alternative of V-based catalysts have become one of the most efficient catalysts in SCR catalysis due to the Fe2+/Fe3+ redox circle [74,75]. The development of Fe-based catalysts generally focused on the control of the morphology and structure as well as the modification of the catalyst surface. The overoxidation of NH3 under high temperature is another concern for Fe-based catalysts. Wang et al. [49] successfully synthesized WO3-FeOx catalysts by a solvent-free method. The introduction of WO3 restrained the growing of the Fe2O3 particles and inhibited the agglomeration of the particles. And the NOx conversion over the catalyst could achieve 90% between 200 ℃ and 500 ℃. Liu et al. [76] also found that the addition of WOx could improve the activity of Fe2O3 catalyst by supplying abundant surface reactive Lewis and Bronsted acid sites. The NOx conversion could achieve 80% above from 300 ℃ to 450 ℃.
Han et al. [77] anchored Fe2O3–CeO2 onto the mesoporous Al2O3 nanoarrays which were in situ created on the surface of Al-mesh. The Al2O3 nanoarrays supplied abundant sites for the anchoring of metal oxides catalysts. Furthermore, they also founded that the presence of Ce changed the charge distribution of Fe and the interaction between Ce and Fe benefited NH3-SCR. Chen et al. [65] Introduce the single-atom Ce species to the surface of α-Fe2O3 to improve the content of Fe2+ and decrease the oxygen vacancy formation energy. NO conversation over the Ce-modified α-Fe2O3 was above 95% at a very low temperature of 175 ℃.
2.1.3 Metal ion-exchanged zeolite catalysts
The ion-exchanged zeolites are another high-efficiency substitute of traditional V2O5/TiO2 catalysts. In recent years, Cu-based exchanged zeolites have been the most widely studied zeolite catalyst in NOx removal because its active temperature window could cover low, medium and high temperature [18,20,78–83].
Sun et al. [84] investigated the influence of Si/Al ratio on NH3-SCR activity of Cu-SSZ-13 zeolite, and found that the Si/Al ratio or Cu loadings could influence the hydrothermal stability and catalytic activity of Cu-SSZ-13 zeolite by controlling the formation of Cu2+–2Al and [CuⅡ(OH)]+–Al. Jin et al. [62] reported that the existence of Cu species on zeolite surface could promote the adsorption and activation of NH3. And their NH3-SCR activity test result indicated that the T90 (temperature window of NOx conversion ≥ 90%) temperature window was 200–350 ℃. Daya et al. [85] developed the kinetic model of the CuⅠ/CuⅡ redox circle during NH3-SCR. Wang et al. [86] prepared Cu-ZSM-5 catalysts with different morphologies (nanosheets, nanoparticles and hollow spheres). They found that the NO conversion over nanosheet-like Cu-ZSM-5 (NS-Cu-CZSM-5) could achieved nearly 100% in the temperature window from 200 ℃ to 400 ℃. Furthermore, they also found that Cu-based zeolite contains CuO and CuO is bad for NO reduction. Several strategies have been reported in inhibiting the growth of CuO crystal. Zhang et al. [87] suppressed the generation of CuO by increasing the amounts of acidic sites in zeolite structure. They widened the T90 of Cu exchanged zeolite to 200–600 ℃. Ma et al. [88] reported that the formation of CuO in Cu-SSZ could be restrained by surface Al modification. And the T90 temperature range could be widened to 200–500 ℃. Yue et al. [57] successfully restrained the generation and growth of CuO grains by Fe modification. And the NO conversion over FeCu-SSZ-13 could achieved above 95% within the temperature range of 200–550 ℃. Except for Cu, some metal ions such as Fe and Ce also are attractive compensation ion for zeolite catalysts. Shi et al. [89] reported the NOx conversion over In and Ce bimetallic catalyst supported by zeolite nearly achieved 100% within the temperature range of 250–600 ℃. Liu et al. [90] reported a Fe-Ni-W zeolite catalyst and investigated its NH3-SCR activity in a wide temperature window of 200 ℃–850 ℃.
In recent years, some researchers are trying to synthesize and develop zeolites with different framework structure as NH3-SCR catalysts. Tarach et al. [80] studied the influence of zeolite topology on NH3-SCR activity and found that topology structure could affect the redox circle between Cu2+ and Cu0. Zhang et al. [91] reported a new CHA-type aluminoborosilicates and applied it to deNOx reaction. The T90 temperature range of the catalyst could be widened to 200–500 ℃. Chitac et al. [92] synthesized a small-pore SWY zeolite and further introduced Cu to zeolite framework structure. The NH3-SCR light-off test indicated that the catalyst could achieve 90% NOx conversion between 200 ℃ and 500 ℃.
In conclusion, in consideration of vanadium biotoxicity, developing novel vanadium-free catalysts working in a wide active temperature window has been a research hotspot. The critical factors which affect the performance of SCR catalysts are acid site strength and redox properties. The general strategies to improve acid site strength and redox property included doping modification, grain growth control and dominant crystal planes exposure. In recent years, some novel NH3-SCR catalysts such as Fe-, Ce-based catalysts have been widely reported. However, the synthesis methods of these catalysts involve fancy instruments and require accurate parameter control. As a result, how to simplify the preparation procedures of the catalysts is critical for the mass production of novel vanadium-free deNOx catalysts.
2.2 Improve the SO2 resistance of SCR catalysts
Many studies have shown that the presence of SO2 in the flue gas from coal-fired plants negatively influence the catalytic activity of SCR catalysts. The negative effects of SO2 on SCR catalysts include physical deactivation and chemical deactivation. The physical deactivation was caused by the deposition of NH4HSO4 (ABS) over catalysts surface [32] while the chemical deactivation was the sulfation of active sites. In particular, the physical deactivation could be the main problem when the working temperature of deNOx was below 300 ℃. ABS becomes a very viscous liquid (0.1–0.2 Pa·s) within the temperature range of 147–350 ℃ [93,94] and sticks to the surface of catalysts with coal ash, leading to the reactor channel being blocked. As described in Fig. 3, the generation of ABS proceed via three main steps: the adsorption of SO2, the oxidation of SO2 and the binding of NH4+ and HSO4−. The general strategies to improve the SO2 resistance of catalysts focused on inhibiting the adsorption and oxidation of SO2 and promoting the decomposition of ABS. In Table 3, We made a summary about the strategies to improve the SO2 resistance of NH3-SCR catalysts.
Figure 3
Table 3
2.2.1 Inhibiting the adsorption of SO2
Inhibiting the adsorption of SO2 on the surface of catalysts was an important strategy to directly curb the generation of ABS [95–98]. The SO2 adsorption could be inhibited by introducing some special catalytic promoters, constructing sacrificial sites, creating protection layer and so on. The catalytic promoter which could strengthen the acidity or weaken the alkalinity of active sites is beneficial for inhibiting SO2 adsorption because SO2 is atypical acidic oxide. Some nonmetal elements or metal elements with strong electronegativity are always good options. Kang et al. [99] found that the introduction of Fe into CeVO4 could restrain the adsorption of SO2 by increasing the types and amounts of acidic sites. The long-term NH3-SCR test in the presence of 100 ppm SO2 showed that the NO conversion over FeδCe1-δVO4 could maintain above 80% after 25 h. Tan et al. [100] successfully synthesized innovative CeO2-SiO2 mix oxide using coprecipitation method. The strong interaction of Ce-O-Si weaken the alkalinity of CeO2 and further inhibited the adsorption of SO2 on Ce. The catalytic stability tests in the presence of 500 ppm SO2 indicated that the NO conversation over CeO2–SiO2 could still maintain about 90% after 40 h aging. Chen [101] introduced Co species into MnOx and found that the NOx conversion over Co-modified MnOx could be kept at above 85% in the presence of 50 ppm SO2 for 10 h (Fig. 4a). Park et al. [102] introduced Nb and Mo to VOx-oxide and kept NOx conversion above 80% after 124 h in the presence of 500 ppm SO2.
Figure 4
Figure 4. Schematic illustration of (a, b) inhibiting adsorption of SO2. Reprinted with permission [101]. Copyright 2023, Elsevier. Reprinted with permission [103]. Copyright 2022, American Chemical Society. (c, d) inhibiting oxidation of SO2. Reprinted with permission [105]. Copyright 2021, American Chemical Society. Reprinted with permission [106]. Copyright 2020, American Chemical Society. (e, f) promoting regeneration of active sites. Reprinted with permission [36]. Copyright 2021, Elsevier. Reprinted with permission [114]. Copyright 2018, American Chemical Society.Moreover, creating protective layer or introducing sacrificial agents could also protect metallic active sites from the harm of SO2 adsorption. Qi et al. [103] deposited a layer of iron sulfate on the surface of CeO2/TiO2 catalyst. The presence of surface iron sulfate cut off SO2 adsorption pathway and inhibited the sulfation of Ce species (Fig. 4b). Fang et al. [104] doped Ce atoms into MnO2 as the sacrificial sites of SO2 adsorption and oxidation. Furthermore, they found that the generation of Ce2(SO4)3 could supply extra adsorption sites for NH4+ to upgrade the reaction of NOx reduction. Tang [97] prepared a protect layer of amorphous MoOx on the surface of CeFeOx and the NOx conversion could maintain above 90% in the presence of SO2 for 60 h.
2.2.2 Inhibiting the oxidation of SO2
The SO2 resistance of catalysts could also be improved by inhibiting the oxidation of SO2 [105–109]. The key to restrain SO2 oxidation is suppressing the electron transfer from SO2 to metal active centers. Some researchers are trying to confining active metal species on nanoscale catalyst support to achieve this goal. Hu et al. [105] successfully prepared highly dispersed ceria particles by loading CeO2 onto the surface of MoO3 nanorod support. And the percentage of Ce3+ increased from 9% (bulk CeO2) to 60% (CeO2/MoO3) (Fig. 4c). The catalyst could maintain nearly 100% NO conversion in the presence of 1000 ppm SO2 for 20 h at 280 ℃. Xu et al. [110] confined active Cu species in TiO2 nanotubes and successfully improve the Cu+/Cu2+ ratio. The NOx conversion of the catalyst could keep above 80% after 100 ppm SO2 poisoning test of 8 h. Wang et al. [111] synthesized Ce-based catalyst which used montmorillonite/TiO2 composite as the support. The NO conversion over the catalyst still maintained above 80% in the presence of 100 ppm SO2 after 5 h test.
Furthermore, constructing facile electron transfer pathway by introducing electron donor could also suppress the electron transfer between SO2 and active metal sites. Wang et al. [106] doped Sm into MnCeTiOx catalyst to inhibit SO2 oxidation by Mn4+ and Ce4+. The electron transfer pathways of Sm2+ → Mn4+ and Sm2+ → Ce4+ suppressed the electron transfer between SO2 and Mn4+/Ce4+ (Fig. 4d). Purbia et al. [107] doped CuO—CeO2 nano-heterostructures to V2O5–CeO2–WO3/TiO2 catalysts to improve SO2 resistance of V2O5-based catalysts. They found that the facile electron transfer between Cu-Ce and V restrained the oxidation of SO2 on the surface of active sites. Xu et al. [112] prepared a MnCe/GAC—CNTs catalyst with excellent SO2 resistance. And the electron transfer between Ce and Mn restrained the oxidation of SO2.
2.2.3 Promoting the regeneration of catalytic active sites
Because the decomposition temperature of ABS was above 350 ℃, the deposition of ABS on catalyst surface was unavoidable while the system was running below 300 ℃. Removing ABS from catalyst surface was critical for the regeneration of active sites covered by ABS. The general strategies are promoting the decomposition of ABS or inducing the migration of ABS [37,113–115].
Kwon et al. [36] found that the decomposition of ABS/AS was related with the oxygen vacancies and reactive oxygen species in catalysts (Fig. 4e). They added Sb-Ce to V2O5/TiO2 and found that the introduction of Sb-Ce could improve the ratio of unstable Oα species. And further the decomposition temperature of ABS would decrease from 300 ℃ above to 264 ℃. Chen [114] successfully synthesized Fe2O3 on the surface of α-MoO3 nanobelts (Fig. 4f). The α-MoO3 with the structure of nanobelt could trap NH4+ from ABS and promote the decomposition of ABS. Guo et al. [116] found that MoO3 could capture NH4+ to promote the decomposition of ABS. Guo et al. successfully prepared sub-monolayer MoO3 on the surface of TiO2 and found that the atomically dispersed O-Mo-O could effectively improve the decomposition of ABS on TiO2. Chen et al. [117] prepared a TiO2-support single-atom Mo catalyst and successfully decreased the decomposition temperature of ABS to 225 ℃.
Inducing the migrant of ABS is another effective strategy to remove the ABS on catalyst surface. Song et al. [118] physically mixed H-Y zeolite with V2O5/TiO2 and used H-Y zeolite as a trapper of ABS to protect vanadium active sites. The regeneration and reusability test indicated that the VWTi + Z catalyst aged for 20 h by SO2 could achieve almost the same performance with fresh catalyst after regeneration at 350 ℃. Jeon et al. [27] used alumina as the trapper of ABS to protect V2O5/WO3-TiO2 catalyst. And the catalyst could almost totally regeneration after calcining at 350 ℃.
Inhibiting AS/ABS deposition is critical to improve SO2 resistance of catalysts at low temperature below 300 ℃. The general strategies adopted in previous studies were cutting off the generation pathway of AS/ABS and removing AS/ABS from catalyst surface. However, flue gas is a complex mixture which include water vapor, CO2, particulates, alkali/alkaline/heavy metals, SO2 and NOx. And there are still lack of related studies about the poisoning mechanism of muti-pollutants coexistence. It is important for the developing of NH3-SCR which have excellent poisoning resistance.
3. Recent progress of SCR catalyst in municipal waste incineration
3.1 Ultra-low temperature SCR catalysts (≤ 200 ℃)
As a promising substitute of traditional power generation, biomass combustion power generation has gained increasing attention. The municipal waste incineration as a source of renewable biomass energy is gradually becoming an important power generation technology. However, the deNOx treatment of flue gas in municipal waste incineration power plants restrained its development. The temperature of flue gas from municipal waste incineration power plants generally ranges between 100 ℃ and 200 ℃, and can not reach the ignition temperature of traditional V2O5-based catalysts. Compared with other types of deNOx catalysts, MnOx-based catalysts could exhibit excellent deNOx efficiency under ultra-low temperature condition (≤ 200 ℃) [119–122]. Table 4 summarizes the NH3-SCR catalysts working under ultra-low temperature condition. The modifications of MnOx catalysts include structure or morphology modification of single MnOx, construction of MnOx-based composite oxides and the construction of core-shell structure [78,114,119,120,123–130].
Table 4
The crystallinity is one of the most important factors which affect the activity of catalysts. The low crystallinity benefited the generation of oxygen defects on catalysts. Therefore, most studies focused on decreasing the crystallinity of MnO2 to improve the catalytic activity of MnO2. Yang et al. [125] successfully reduced the crystallinity of MnO2 by a facile procedure of phase transformation. According to the HRTEM and XPS results of β-MnO2, the considerable amounts of defects and the high concentrations of surface absorbed oxygen were detected, indicating that the concentrations of oxygen defects were increased in β-MnO2 compared with that in γ-MnO2. Furthermore, NO conversation over β-MnO2 could maintain almost 100% NO conversation when the working temperature was decreased to 150 ℃. Zhang [126] successfully synthesized an amorphous MnOx by in-situ deposition as the catalyst of deNOx reaction, which exhibited excellent performance for low temperature deNOx.
However, the working temperature windows of single MnOx catalysts are very narrow. Lots of efforts have been devoted to broaden the working temperature windows of MnOx catalysts. Construction of MnOx-based composite oxides was an effective method to widen the working temperature of MnOx. Some transition or lanthanide metals (Ce, Fe, Sm, Gd, etc.) have been introduced to combine with MnOx. Wang [131] introduced Sm to MnOx and subsequently erased Sm atoms from SmMnOx by nitric acid. They found that the removal of Sm led to the exposure of Mn-O and Mn-Mn dual-functional sites and the increased Mn4+/Mn3+ ratio (Figs. 5a and b). They also found that the NO conversation over SmMnO5 after being treated by nitric acid could maintain about 100% in a very wide temperature window from 80 ℃ to 210 ℃. Xu et al. [132] found doping Ti and Sm to MnOx widened the optimal working temperature from 170–210 ℃ to 70–230 ℃. Geng et al. [127] successfully prepared W-Mn3CeOx which showed a good SCR activity at the temperature of 120–260 ℃.
Figure 5
Figure 5. Schematic illustration of (a, b) the NH3-SCR reaction over the SM-E catalyst. Reprinted with permission [131]. Copyright 2022, American Chemical Society.In conclusion, Mn-based catalyst has become one of the most potential catalysts which could directly catalyze NH3-SCR process under ultra-low temperature condition. The studies about widening active temperature window and improving N2 selectivity of MnOx-based catalysts have made a great progress in recent years. However, a lot of components including alkali/alkaline-earth/heavy metals (Mg, K, Na, Hg, etc.) and acidic compounds (HCl, HF, etc.), abundantly existed in the flue gas could cause the deactivation of Mn-based catalyst. Improving the poison resistance of Mn-based catalyst can be the focus of future studies.
3.2 SO2 + H2O resistance
The deNOx catalysts composed by MnOx-based oxides always show terrible stability in the presence of H2O and SO2. However, the combustion of municipal waste incineration produces a large number of water vapor (≥ 25%) and SO2. MnOx-based oxides has become one of the most promising deNOx catalysts in municipal waste incineration industry. How to improve the stability of MnOx-based catalysts in the presence of H2O + SO2 was another urgent issue which need be tackled for the development of deNOx catalysts in municipal waste incineration industry [2,104,133–135]
Fig. 6 shows the deactivation mechanism of deNOx catalysts in the presence of H2O + SO2. Water vapor can compete for active sites with reactants and aggravate the poisoning effect of SO2.
Figure 6
The general strategies to improve H2O + SO2 resistance of MnOx catalysts are also described in Fig. 6. Introducing sacrificial or protective layer was one of the most effective strategies to inhibit the influence of H2O + SO2. Huang et al. [63] constructed a TiO2 nanocage on the surface of CeMnOx by directional hydrolytic etching of MIL-125 (Fig. 7a). The as-prepared CeMnOx exhibited excellent water vapor resistance under the protection of TiO2 nanocages. Song et al. [136] also achieved protective effect by introducing Ce-Ti as the sacrificial sites of H2O and SO2 adsorption. Furthermore, improving the amounts of active sites was another effective strategy [134]. Raja et al. [137] modified Mn/TiO2 catalyst by the introducing of CNTs and Cu. Their results demonstrated that the incorporation of CNTs and Cu increased the amount and acidity of active sites on the MnOx. In addition, fabricating special structures or optimizing the pore channel of catalysts could also improve the H2O + SO2 resistance of deNOx catalysts. Shi et al. [134] successfully synthesized a MnCoOx catalyst with the unique hollow nanotube structure (Fig. 7b). They found that the unique structure of catalyst well protected the active sites in the inner surface from the poisoning of H2O + SO2. The NOx conversion over MnCoOx could maintain above 80% for a long time in the presence of 10% H2O and 100 ppm SO2.
Figure 7
All in all, the general strategies for improving H2O + SO2 resistance of Mn-based catalyst focused on inhibiting the adsorption of vaporous H2O for now. However, the liquefaction of water vapor would happen with the decrease of working temperature. And the liquid film of H2O would cover the surface of catalyst and cut off reaction pathway. Therefore, in order to apply the Mn-based catalyst in the industrial practices in the future, the new problems caused by the state change of H2O will be a concern.
4. Conclusion and perspective
In conclusion, this review discussed the challenges SCR catalysts are facing in Coal-fired power plants which operate under low-load conditions and SCR catalysts are facing in municipal waste incineration. The studies on broadening working temperature range and improving the SO2 resistance of SCR catalysts are summarized in coal-fired power plants which operate under low-load conditions and in municipal waste incineration comprehensively.
Although the studies on SCR catalysts have made a great progress, there are still some research directions which should be focused on:
(1) It is generally accepted that the reaction pathway of NH3-SCR is controlled by L-H and E-R mechanism. However, which mechanism plays a leading role at specific working temperature have not been identified. In the future, the reaction mechanisms of NH3-SCR should be studied at different working temperature. The related mechanism studies are the guidance of developing novel SCR catalysts which are adapted to specific working temperature.
(2) For now, the industrial application of powder NH3-SCR catalysts is generally achieved by assembling monolithic catalysts. The main strategy is to coat catalysts on the surface of base materials. However, there are some problems which restrain the further development of monolithic catalysts in industry. The low utilization rate of catalyst coating results in limited deNOx efficiency. Furthermore, the catalyst coating is easily to drop off due to low interface strength between powder catalysts and supports. These problems have been ignored during the development of catalysts for a long time. The following research directions are deserved to be focused on: how to choose suitable base materials and control the interface between base and catalyst coating; how to design and optimize the structure and reaction channel; the mass and heat transfer studies of the whole reactors; the construction of simulation models for screening and optimizing monolithic structure.
(3) Considering the complex components of industry flue gas, how to improve the catalytic performance of catalysts in terms of multi-pollutants degradation is a prospective research direction. Developing novel catalysts which could efficiently eliminate the multi-pollutants simultaneously is the optimal strategy to simplify the devices and decrease the costs.
Declaration of competing interest
The authors declare that they have no known competing financial interests or personal relationships that could have appeared to influence the work reported in this paper.
Acknowledgments
This work was financially supported by the National Natural Science Foundation of China (No. 52072306) and the National Key R&D Program of China (No. 2021YFB3701500), and the Fundamental Research Funds for the Central Universities (Nos. 3102019PJ008 and 3102018JCC002).
-
-
[1]
Y. Liu, L. Liu, Y. Wang, Environ. Sci. Technol. 55 (2021) 9691–9710. doi: 10.1021/acs.est.1c01531
-
[2]
G. Xu, X. Guo, X. Cheng, et al., Nanoscale 13 (2021) 7052–7080. doi: 10.1039/D1NR00248A
-
[3]
L. Zhang, Y. Wang, C. Feng, et al., Sci. Total Environ. 770 (2021) 145242. doi: 10.1016/j.scitotenv.2021.145242
-
[4]
Y. Huang, M. Zhu, M. Ji, et al., Am. J. Respir. Crit. Care Med. 204 (2021) 817–825. doi: 10.1164/rccm.202011-4063OC
-
[5]
A. Bettiol, E. Gelain, E. Milanesio, et al., Environ. Health 20 (2021) 46. doi: 10.1186/s12940-021-00728-9
-
[6]
Z. Khorrami, M. Pourkhosravani, M. Rezapour, et al., Sci. Rep. 11 (2021) 9239. doi: 10.1038/s41598-021-88643-4
-
[7]
X. Lu, T. Yao, Y. Li, et al., Environ. Pollut. 212 (2016) 135–146. doi: 10.1016/j.envpol.2016.01.056
-
[8]
Z. Zong, C. Tian, J. Li, et al., Environ. Sci. Technol. 54 (2020) 7787–7797. doi: 10.1021/acs.est.0c00490
-
[9]
Z. Zong, X. Wang, C. Tian, et al., Environ. Sci. Technol. 51 (2017) 5923–5931. doi: 10.1021/acs.est.6b06316
-
[10]
X. Bo, M. Jia, X. Xue, et al., Nat. Sustain. 4 (2021) 811–820. doi: 10.1038/s41893-021-00736-0
-
[11]
L. Li, C. Tang, X. Cui, et al., Angew. Chem. Int. Ed. 60 (2021) 14131–14137. doi: 10.1002/anie.202104394
-
[12]
H. Liu, J. Park, Y. Chen, et al., ACS Catal. 11 (2021) 8431–8442. doi: 10.1021/acscatal.1c01525
-
[13]
K. Masera, A.K. Hossain, Fuel 298 (2021) 120826. doi: 10.1016/j.fuel.2021.120826
-
[14]
D. Wang, Q. Chen, X. Zhang, et al., Environ. Sci. Technol. 55 (2021) 2743–2766. doi: 10.1021/acs.est.0c07326
-
[15]
G. Song, Y. Xiao, Z. Yang, et al., Fuel 292 (2021) 120276. doi: 10.1016/j.fuel.2021.120276
-
[16]
A. Talaiekhozani, S. Rezania, K.H. Kim, et al., J. Clean. Prod. 278 (2021) 123895. doi: 10.1016/j.jclepro.2020.123895
-
[17]
Y.W. Wu, X.Y. Zhou, J.L. Zhou, et al., Sci. Total Environ. 857 (2023) 159712. doi: 10.1016/j.scitotenv.2022.159712
-
[18]
T. Andana, K.G. Rappé, F. Gao, et al., Appl. Catal. B 291 (2021) 120054. doi: 10.1016/j.apcatb.2021.120054
-
[19]
B. Ye, B. Jeong, M.J. Lee, et al., Nano Converg. 9 (2022) 51. doi: 10.1186/s40580-022-00341-7
-
[20]
Y. Shan, J. Du, Y. Zhang, et al., Natl. Sci. Rev. 8 (2021) nwab010. doi: 10.1093/nsr/nwab010
-
[21]
W. Shan, H. Song, Catal. Sci. Technol. 5 (2015) 4280–4288. doi: 10.1039/C5CY00737B
-
[22]
W. Shan, Y. Yu, Y. Zhang, et al., Catal. Today 376 (2021) 292–301. doi: 10.1016/j.cattod.2020.05.015
-
[23]
G. Busca, L. Lietti, G. Ramis, et al., Appl. Catal. B 18 (1998) 1. doi: 10.1016/S0926-3373(98)00040-X
-
[24]
I. Malpartida, O. Marie, P. Bazin, et al., Appl. Catal. B 113-114 (2012) 52–60.
-
[25]
Z. Lian, J. Wei, W. Shan, et al., J. Am. Chem. Soc. 143 (2021) 10454–10461. doi: 10.1021/jacs.1c05354
-
[26]
G. Xu, H. Li, Y. Yu, et al., Environ. Sci. Technol. 56 (2022) 3710–3718. doi: 10.1021/acs.est.1c07739
-
[27]
S.W. Jeon, I. Song, H. Lee, et al., Chem. Eng. J. 433 (2022) 133836. doi: 10.1016/j.cej.2021.133836
-
[28]
Z. Lian, L. Liu, C. Lin, et al., Environ. Sci. Technol. 56 (2022) 9744–9750. doi: 10.1021/acs.est.2c02395
-
[29]
J. Cao, C. Nannuzzi, W. Liu, et al., Fuel 328 (2022) 125262. doi: 10.1016/j.fuel.2022.125262
-
[30]
S. Xiong, J. Chen, H. Liu, et al., Environ. Sci. Technol. 56 (2022) 3739–3747. doi: 10.1021/acs.est.2c00113
-
[31]
G. Msigwa, J.O. Ighalo, P.S. Yap, Sci. Total Environ. 849 (2022) 157755. doi: 10.1016/j.scitotenv.2022.157755
-
[32]
K. Guo, J. Ji, W. Song, et al., Appl. Catal. B 297 (2021) 120388. doi: 10.1016/j.apcatb.2021.120388
-
[33]
K. Guo, J. Ji, R. Osuga, et al., Appl. Catal. B 287 (2021) 119982. doi: 10.1016/j.apcatb.2021.119982
-
[34]
Y. Yu, W. Tan, D. An, et al., Appl. Catal. B 281 (2021) 119544. doi: 10.1016/j.apcatb.2020.119544
-
[35]
Z. Zhang, R. Li, M. Wang, et al., Appl. Catal. B 282 (2021) 119542. doi: 10.1016/j.apcatb.2020.119542
-
[36]
D.W. Kwon, D.H. Kim, S. Lee, et al., Appl. Catal. B 289 (2021) 120032. doi: 10.1016/j.apcatb.2021.120032
-
[37]
K. Guo, G. Fan, D. Gu, et al., ACS Appl. Mater. Interfaces 11 (2019) 4900–4907. doi: 10.1021/acsami.8b15688
-
[38]
A.V. Shah, V.K. Srivastava, S.S. Mohanty, et al., J. Environ. Chem. Eng. 9 (2021) 105717. doi: 10.1016/j.jece.2021.105717
-
[39]
A.T. Hoang, P.S. Varbanov, S. Nižetić, et al., J. Clean. Prod. 359 (2022) 131897. doi: 10.1016/j.jclepro.2022.131897
-
[40]
N. Kundariya, S.S. Mohanty, S. Varjani, et al., Bioresour. Technol. 342 (2021) 125982. doi: 10.1016/j.biortech.2021.125982
-
[41]
S. Nanda, F. Berruti, J. Hazard. Mater. 403 (2021) 123970. doi: 10.1016/j.jhazmat.2020.123970
-
[42]
S. Khan, R. Anjum, S.T. Raza, et al., Chemosphere 288 (2022) 132403. doi: 10.1016/j.chemosphere.2021.132403
-
[43]
T. Rasheed, M.T. Anwar, N. Ahmad, et al., J. Environ. Manag. 287 (2021) 112257. doi: 10.1016/j.jenvman.2021.112257
-
[44]
T. Andana, K. Rappé, F. Gao, et al., Appl. Catal. B 291 (2021) 120054. doi: 10.1016/j.apcatb.2021.120054
-
[45]
J. Wang, H. Zhao, G. Haller, et al., Appl. Catal. B 202 (2017) 346–354. doi: 10.1016/j.apcatb.2016.09.024
-
[46]
S. Zhao, J. Peng, R. Ge, et al., Fuel Process. Technol. 236 (2022) 107432. doi: 10.1016/j.fuproc.2022.107432
-
[47]
L. Han, S. Cai, M. Gao, et al., Chem. Rev. 119 (2019) 10916–10976. doi: 10.1021/acs.chemrev.9b00202
-
[48]
S. Liu, H. Wang, Y. Wei, et al., ACS Appl. Mater. Interfaces 11 (2019) 22240–22254. doi: 10.1021/acsami.9b03429
-
[49]
H. Wang, P. Ning, Y. Zhang, et al., J. Hazard. Mater. 388 (2020) 121812. doi: 10.1016/j.jhazmat.2019.121812
-
[50]
W. Zhao, S. Dou, K. Zhang, et al., Chem. Eng. J. 364 (2019) 401–409. doi: 10.1016/j.cej.2019.01.166
-
[51]
P. Gong, J. Xie, D. Fang, et al., Chem. Eng. J. 356 (2019) 598–608. doi: 10.1016/j.cej.2018.09.042
-
[52]
J. Zhang, Z. Huang, Y. Du, et al., Chem. Eng. J. 381 (2020) 122668. doi: 10.1016/j.cej.2019.122668
-
[53]
Y. Pan, B. Shen, L. Liu, et al., Fuel 282 (2020) 118834. doi: 10.1016/j.fuel.2020.118834
-
[54]
S. Ma, X. Zhao, Y. Li, et al., Appl. Catal. B 248 (2019) 226–238. doi: 10.1016/j.apcatb.2019.02.015
-
[55]
Y. Shan, J. Du, Y. Yu, et al., Appl. Catal. B 266 (2020) 118655. doi: 10.1016/j.apcatb.2020.118655
-
[56]
Y. Li, W. Liu, R. Yan, et al., Appl. Catal. B 268 (2020) 118455. doi: 10.1016/j.apcatb.2019.118455
-
[57]
Y. Yue, B. Liu, P. Qin, et al., Chem. Eng. J. 398 (2020) 125515. doi: 10.1016/j.cej.2020.125515
-
[58]
J. Shi, Y. Zhang, Y. Zhu, et al., J. Catal. 378 (2019) 17–27. doi: 10.1016/j.jcat.2019.08.003
-
[59]
Z. Huang, S. Guo, W. Chen, et al., Chem. Eng. J. 464 (2023) 142540. doi: 10.1016/j.cej.2023.142540
-
[60]
P. Gong, J. Xie, D. Fang, et al., Appl. Surf. Sci. 505 (2020) 144641. doi: 10.1016/j.apsusc.2019.144641
-
[61]
Y. Xin, N. Zhang, X. Wang, et al., Catal. Today 332 (2019) 35–41. doi: 10.1016/j.cattod.2018.08.018
-
[62]
Q. Jin, D. Fang, Y. Ye, et al., Appl. Surf. Sci. 600 (2022) 154075. doi: 10.1016/j.apsusc.2022.154075
-
[63]
X. Huang, F. Dong, G. Zhang, et al., Chem. Eng. J. 432 (2022) 134236. doi: 10.1016/j.cej.2021.134236
-
[64]
Y. Li, H. Chen, L. Chen, et al., Appl. Catal. B 318 (2022) 121779. doi: 10.1016/j.apcatb.2022.121779
-
[65]
W. Chen, S. Yang, H. Liu, et al., Environ. Sci. Technol. 56 (2022) 10442–10453. doi: 10.1021/acs.est.2c02916
-
[66]
Z. Wang, J. Lan, M. Haneda, et al., Catal. Today 376 (2021) 222–228. doi: 10.1016/j.cattod.2020.05.040
-
[67]
S. Liu, P. Yao, Q. Lin, et al., Catal. Today 382 (2021) 34–41. doi: 10.1016/j.cattod.2021.05.007
-
[68]
J. Wan, J. Chen, R. Zhao, et al., J. Environ. Sci. China 100 (2021) 306–316. doi: 10.1016/j.jes.2020.08.003
-
[69]
X. Wei, Q. Ke, H. Cheng, et al., Chem. Eng. J. 391 (2020) 123491. doi: 10.1016/j.cej.2019.123491
-
[70]
W. Zhao, K. Zhang, L. Wu, et al., J. Colloid Interface Sci. 581 (2021) 76–83. doi: 10.1016/j.jcis.2020.07.131
-
[71]
J. Cao, X. Yao, F. Yang, et al., Chin. J. Catal. 40 (2019) 95–104. doi: 10.1016/S1872-2067(18)63184-5
-
[72]
W. Si, H. Liu, T. Yan, et al., Appl. Catal. B 269 (2020) 118797. doi: 10.1016/j.apcatb.2020.118797
-
[73]
I. Song, S. Youn, H. Lee, et al., Appl. Catal. B 210 (2017) 421–431. doi: 10.1016/j.apcatb.2017.04.016
-
[74]
C. Sun, W. Chen, X. Jia, et al., Chin. J. Catal. 42 (2021) 417–430. doi: 10.1016/S1872-2067(20)63666-X
-
[75]
J. Liu, H. Cheng, H. Zheng, et al., ACS Catal. 11 (2021) 14727–14739. doi: 10.1021/acscatal.1c04497
-
[76]
F. Liu, W. Shan, Z. Lian, et al., Appl. Catal. B 230 (2018) 165–176. doi: 10.1016/j.apcatb.2018.02.052
-
[77]
L. Han, M. Gao, C. Feng, et al., Environ. Sci. Technol. 53 (2019) 5946–5956. doi: 10.1021/acs.est.9b01217
-
[78]
H. Kubota, T. Toyao, Z. Maeno, et al., ACS Catal. 11 (2021) 11180–11192. doi: 10.1021/acscatal.1c02860
-
[79]
Y. Xi, N.A. Ottinger, C.J. Keturakis, et al., Appl. Catal. B 294 (2021) 120245. doi: 10.1016/j.apcatb.2021.120245
-
[80]
K.A. Tarach, M. Jabłońska, K. Pyra, et al., Appl. Catal. B 284 (2021) 119752. doi: 10.1016/j.apcatb.2020.119752
-
[81]
Z. Gao, J. Pihl, T. LaClair, et al., Chem. Eng. J. 406 (2021) 127120. doi: 10.1016/j.cej.2020.127120
-
[82]
F. Gramigni, N.D. Nasello, N. Usberti, et al., ACS Catal. 11 (2021) 4821–4831. doi: 10.1021/acscatal.0c05362
-
[83]
J. Liang, J. Tao, Y. Mi, et al., Chem. Eng. J. 409 (2021) 128238. doi: 10.1016/j.cej.2020.128238
-
[84]
Y. Sun, Y. Fu, Y. Shan, et al., Environ. Sci. Technol. 56 (2022) 17946–17954. doi: 10.1021/acs.est.2c03813
-
[85]
R. Daya, D. Trandal, U. Menon, et al., ACS Catal. 12 (2022) 6418–6433. doi: 10.1021/acscatal.2c01076
-
[86]
H. Wang, J. Jia, S. Liu, et al., Environ. Sci. Technol. 55 (2021) 5422–5434. doi: 10.1021/acs.est.0c08684
-
[87]
S. Zhang, S. Ming, L. Guo, et al., J. Hazard. Mater. 414 (2021) 125543. doi: 10.1016/j.jhazmat.2021.125543
-
[88]
Y. Ma, S. Cheng, X. Wu, et al., J. Catal. 405 (2022) 199–211. doi: 10.1016/j.jcat.2021.11.022
-
[89]
Y. Shi, J. Pu, L. Gao, et al., Chem. Eng. J. 403 (2021) 126394. doi: 10.1016/j.cej.2020.126394
-
[90]
H. Liu, C. You, H. Wang, ACS Catal. 11 (2021) 1189–1201. doi: 10.1021/acscatal.0c03949
-
[91]
J. Zhang, P. Ji, L. Ren, et al., Chem. Eng. J. 444 (2022) 136657. doi: 10.1016/j.cej.2022.136657
-
[92]
R.G. Chitac, J. Bradley, N.D. McNamara, et al., Chem. Mater. 33 (2021) 5242–5256. doi: 10.1021/acs.chemmater.1c01329
-
[93]
H. Liu, C. Sun, Z. Fan, et al., Catal. Sci. Technol. 9 (2019) 3554–3567. doi: 10.1039/C9CY00731H
-
[94]
H. Liu, Z. Fan, C. Sun, et al., Appl. Catal. B 244 (2019) 671–683. doi: 10.1016/j.apcatb.2018.12.001
-
[95]
L. Li, P. Li, W. Tan, et al., Chin. J. Catal. 41 (2020) 364–373. doi: 10.1016/S1872-2067(19)63437-6
-
[96]
C. Liu, L. Chen, J. Li, et al., Environ. Sci. Technol. 46 (2012) 6182–6189. doi: 10.1021/es3001773
-
[97]
X. Tang, Y. Shi, F. Gao, et al., Chem. Eng. J. 398 (2020) 125619. doi: 10.1016/j.cej.2020.125619
-
[98]
F. Wang, B. Shen, S. Zhu, et al., Fuel 249 (2019) 54–60. doi: 10.1016/j.fuel.2019.02.113
-
[99]
L. Kang, L. Han, P. Wang, et al., Environ. Sci. Technol. 54 (2020) 14066–14075. doi: 10.1021/acs.est.0c05038
-
[100]
W. Tan, A. Liu, S. Xie, et al., Environ. Sci. Technol. 55 (2021) 4017–4026. doi: 10.1021/acs.est.0c08410
-
[101]
R. Chen, X. Fang, J. Li, et al., Chem. Eng. J. 452 (2023) 139207. doi: 10.1016/j.cej.2022.139207
-
[102]
Y. Jae Park, D. Ho Kim, J.H. Lee, et al., Appl. Surf. Sci. 614 (2023) 156072. doi: 10.1016/j.apsusc.2022.156072
-
[103]
X. Qi, L. Han, J. Deng, et al., Environ. Sci. Technol. 56 (2022) 5840–5848. doi: 10.1021/acs.est.2c00944
-
[104]
X. Fang, Y. Liu, Y. Cheng, et al., ACS Catal. 11 (2021) 4125–4135. doi: 10.1021/acscatal.0c05697
-
[105]
X. Hu, J. Chen, W. Qu, et al., Environ. Sci. Technol. 55 (2021) 5435–5441. doi: 10.1021/acs.est.0c08736
-
[106]
B. Wang, M. Wang, L. Han, et al., ACS Catal. 10 (2020) 9034–9045. doi: 10.1021/acscatal.0c02567
-
[107]
R. Purbia, S.Y. Choi, H.J. Kim, et al., Chem. Eng. J. 437 (2022) 135427. doi: 10.1016/j.cej.2022.135427
-
[108]
Z. Chen, S. Ren, M. Wang, et al., Fuel 321 (2022) 124113. doi: 10.1016/j.fuel.2022.124113
-
[109]
X. Li, Y. Niu, J. Li, et al., Chem. Eng. J. 454 (2023) 140180. doi: 10.1016/j.cej.2022.140180
-
[110]
Z. Xu, S. Impeng, X. Jia, et al., Environ. Sci. Nano 9 (2022) 2121–2133. doi: 10.1039/D2EN00144F
-
[111]
Z. Wang, M. Jiao, Z. Chen, et al., Microporous Mesoporous Mater. 320 (2021) 111072. doi: 10.1016/j.micromeso.2021.111072
-
[112]
Y. Xu, P. Wang, Y. Pu, et al., Sep. Purif. Technol. 305 (2023) 122498. doi: 10.1016/j.seppur.2022.122498
-
[113]
J. Yu, E. Zhang, L. Wang, et al., Energy Fuels 34 (2020) 2107–2116. doi: 10.1021/acs.energyfuels.9b04033
-
[114]
Y. Chen, C. Li, J. Chen, et al., Environ. Sci. Technol. 52 (2018) 11796–11802.
-
[115]
T. Tong, J. Chen, S. Xiong, et al., Catal. Sci. Technol. 9 (2019) 3779–3787. doi: 10.1039/C9CY00900K
-
[116]
S. Guo, Z. Huang, L. Wang, et al., J. Hazard. Mater. 418 (2021) 126289. doi: 10.1016/j.jhazmat.2021.126289
-
[117]
J. Chen, X. Fang, Z. Ren, et al., J. Mater. Chem. A 10 (2022) 6065–6072. doi: 10.1039/D1TA08269H
-
[118]
I. Song, H. Lee, S.W. Jeon, et al., Nat. Commun. 12 (2021) 901. doi: 10.1038/s41467-021-21228-x
-
[119]
L. Yan, Y. Gu, L. Han, et al., ACS Appl. Mater. Interfaces 11 (2019) 11507–11517. doi: 10.1021/acsami.9b01291
-
[120]
Y. Xin, H. Li, N. Zhang, et al., ACS Catal. 8 (2018) 4937–4949. doi: 10.1021/acscatal.8b00196
-
[121]
G. He, M. Gao, Y. Peng, et al., Environ. Sci. Technol. 55 (2021) 6995–7003. doi: 10.1021/acs.est.0c08214
-
[122]
W. Wang, L. Wang, Y. Rao, et al., Appl. Surf. Sci. 618 (2023) 156638. doi: 10.1016/j.apsusc.2023.156638
-
[123]
Y. Shi, H. Yi, F. Gao, et al., J. Hazard. Mater. 413 (2021) 125361. doi: 10.1016/j.jhazmat.2021.125361
-
[124]
H. Chang, X. Chen, J. Li, et al., Environ. Sci. Technol. 47 (2013) 5294–5301. doi: 10.1021/es304732h
-
[125]
R. Yang, S. Peng, B. Lan, et al., Small 17 (2021) e2102408. doi: 10.1002/smll.202102408
-
[126]
X. Zhang, X. Hu, S. Liu, et al., J. Environ. Chem. Eng. 10 (2022) 107318. doi: 10.1016/j.jece.2022.107318
-
[127]
Y. Geng, W. Shan, F. Liu, et al., J. Hazard. Mater. 405 (2021) 124223. doi: 10.1016/j.jhazmat.2020.124223
-
[128]
W. Yoon, Y. Kim, G.J. Kim, et al., Chem. Eng. J. 434 (2022) 134676. doi: 10.1016/j.cej.2022.134676
-
[129]
Z. Liu, G. Sun, C. Chen, et al., ACS Catal. 10 (2020) 6803–6809. doi: 10.1021/acscatal.0c01284
-
[130]
S. Xie, W. Tan, Y. Li, et al., ACS Catal. 12 (2022) 2441–2453. doi: 10.1021/acscatal.1c05661
-
[131]
F. Wang, P. Wang, T. Lan, et al., ACS Catal. 12 (2022) 7622–7632. doi: 10.1021/acscatal.2c01897
-
[132]
Q. Xu, Z. Fang, Y. Chen, et al., Environ. Sci. Technol. 54 (2020) 2530–2538. doi: 10.1021/acs.est.9b06701
-
[133]
X. Shi, J. Guo, T. Shen, et al., Chem. Eng. J. 421 (2021) 129995. doi: 10.1016/j.cej.2021.129995
-
[134]
Y. Shi, H. Yi, F. Gao, et al., Sep. Purif. Technol. 265 (2021) 118517. doi: 10.1016/j.seppur.2021.118517
-
[135]
C. Niu, B. Wang, Y. Xing, et al., J. Clean. Prod. 290 (2021) 125858. doi: 10.1016/j.jclepro.2021.125858
-
[136]
J. Song, S. Liu, Y. Ji, et al., Nano Res. 16 (2022) 299–308.
-
[137]
S. Raja, M.S. Alphin, L. Sivachandiran, et al., Fuel 307 (2022) 121886. doi: 10.1016/j.fuel.2021.121886
-
[1]
-
Figure 4 Schematic illustration of (a, b) inhibiting adsorption of SO2. Reprinted with permission [101]. Copyright 2023, Elsevier. Reprinted with permission [103]. Copyright 2022, American Chemical Society. (c, d) inhibiting oxidation of SO2. Reprinted with permission [105]. Copyright 2021, American Chemical Society. Reprinted with permission [106]. Copyright 2020, American Chemical Society. (e, f) promoting regeneration of active sites. Reprinted with permission [36]. Copyright 2021, Elsevier. Reprinted with permission [114]. Copyright 2018, American Chemical Society.
Figure 5 Schematic illustration of (a, b) the NH3-SCR reaction over the SM-E catalyst. Reprinted with permission [131]. Copyright 2022, American Chemical Society.
Table 1. The summary for composition and temperature of the flue gas in different industries.
Table 2. The summary about the literature data for widen operating temperature windows on NH3−SCR reaction.
Table 3. The summary for the published works which improved SO2 resistance by different strategies.
Table 4. The summary about the performance of catalysts under ultra-low temperature condition.
-

计量
- PDF下载量: 2
- 文章访问数: 339
- HTML全文浏览量: 22