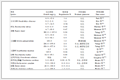

A mini-review of the electro-peroxone technology for wastewaters: Characteristics, mechanism and prospect
English
A mini-review of the electro-peroxone technology for wastewaters: Characteristics, mechanism and prospect
-
1. Introduction
In recent years, harmful trace organic contaminants such as drugs, personal care, and endocrine disruptors have been frequently detected in natural water bodies and water treatment systems [1]. Meanwhile, due to the irrational use and treatment of water resources, a large amount of highly concentrated and hard-to-degrade organic wastewater is discharged into people's daily life. For wastewater with complex and diverse pollutants, poor biochemical properties, and large relative molecular masses, the existing biological treatment methods are often difficult to treat. It is difficult to completely degrade various organic pollutants in wastewater [2]. Advanced oxidation technology is considered to be one of the most effective methods for the removal of organic pollutants [3]. Advanced oxidation technology has been applied in dyeing, chemical, pesticide, electroplating, and other fields [4]. The advanced oxidation processes (AOPs), which are based on the generation of hydroxyl radicals (•OH) with high redox potential, have received much attention [5]. In addition, energy and environment are the most important issues involved in the sustainable development of human society. 80% of the global energy demand comes from fossil fuels, which will eventually lead to the depletion of fossil fuels, and its use will also lead to serious environmental pollution. How to make use of water treatment technology capacity or energy conservation is a problem that needs to be considered in the process of water treatment. For example, hydrogen is one of the ideal clean energy sources and also an important chemical raw material, which has received extensive attention from all countries in the world. In the process of hydrogen production by electrolysis of water, it is an important means to realize industrialization and low-cost hydrogen production. An effective HER electrocatalyst for alkaline water electrolysis based on Mo-Co0.85SeVSe/NC nanosheet has been developed, which can open up many meaningful applications in the electrochemical field, including CO2 reduction, NH3 oxidation, and N2 reduction reactions [6].
The electro-peroxone technology is a novel technology in wastewater treatment in recent years, which makes up for the shortcomings of traditional advanced oxidation technology. The •OH is a non-selective oxidant with a much higher oxidation capacity than ozone (O3). As a coupling technology between electrochemical technology and ozonation technology, the electro-peroxone technology generates hydrogen peroxide (H2O2) through cathodic oxygen (O2) reduction in situ during conventional ozonation. The generated H2O2 can significantly enhance the conversion of O3 to •OH. Carbon-based materials (such as carbon fiber or PTFE electrodes) are often used in the electro-peroxone process. The electro-peroxone process can drive the peroxone reaction electrochemically to degrade pollutants without adding external H2O2 (Eqs. 1 and 2) [7].
(1) (2) The generation of H2O2 from cathodic oxygen reduction is crucial to the success of the electro-peroxide process [8]. However, some other reactions may occur, simultaneously or completely, during the reduction of O2 to H2O2 on the cathode. This depends on the reaction conditions, different processes, and water parameters, such as an electrode, current density, and mixture of O2 and O3. The reduction of O3 may be limited by the applied current or the mass transfer to the cathode of the injected mixture gas of O2 and O3 during the electrolysis process.
This review summarizes the reaction mechanism in the electro-peroxone system from two aspects of bulk reaction and cathodic reaction by analyzing recent research papers. On this basis, it proposes the mechanism of interaction between O3 and cathodic in situ generated H2O2 in this system and the influencing factors of •OH generation. At last, the opportunities and challenges faced by the electro-peroxone technology and its future development direction in wastewater treatment are also proposed.
2. Related advanced oxidation technologies
2.1 Electrochemical oxidation technology
The electrochemical oxidation technology is not only easy to operate, but also has a high degradation rate. It has attracted more and more attention in environmental pollution control, especially in the removal of biodegradable organics from wastewater (Table 1). The electrochemical oxidation treatment of refractory wastewater is the direct electrochemical reaction of pollutants on electrodes or the indirect reaction of pollutants by using strong oxidizing active substances generated on the surface of electrodes to convert pollutants into harmless or less toxic substances through anodic oxidation. The electrochemical oxidation technology is effective in treating refractory and highly toxic wastewater. Common anode materials include Pt, PbO2, SnO2, RuO2 electrodes, etc. The NCNS-CE-PBO2 electrodes have denser surfaces, smaller grains, and many active sites [9]. Furthermore, Xia et al. studied that electrochemical oxidation of AO7 by Fe-doped PbO2 electrode is a promising method, and proposed possible degradation pathways by identifying five kinds of aromatic intermediates for AO7 degradation during the electrochemical process [10]. It also compounded carbon nanotubes into PbO2 electrodes by composite electrodeposition technology and obtained multi-layer CNT-PBO2 electrodes. The PbO2 electrode and CNT-PbO2 electrode were analyzed by SEM and XRD. Considering comprehensively the effects of ISN removal efficiency, COD, and average current efficiency, the ISN degradation rate reached 99.4%, and the COD degradation rate reached 86.8% after the electrode was degraded by electrochemical oxidation for 120 min under the best conditions [11]. A new type of graphene oxide and Yb co-modified PbO2 electrode (Yb GO PbO2) prepared by electrodeposition has good stability, can effectively improve the degradation ability of lamivudine, and has feasibility and good application prospects for the treatment of pharmaceutical wastewater [12]. The decontamination performance of the filter membrane in electrochemical treatment technology is closely related to its electrochemical activity. A novel metal-free composite electrochemical filtration carbon membrane was prepared by deposition of polyaniline layer on CM surface via the facile electro-polymerization oxidation method [13]. At present, the use of three-dimensional electrode is a new electrochemical oxidation technology. Fe/C granules were prepared with iron filings as carrier and sodium alginate as hydrothermal carbonizing agent. Rice husk and iron filings are widely available and inexpensive raw materials for particle electrodes, which use Fe/C particles suspended between the cathode and anode to produce a three-dimensional electrode [14]. The Sn/FCC, Sb/FCC and Sb–SnO2/FCC composites were fabricated via impregnation method [15]. This study provides a new idea for the preparation of electrodes and has a broad application prospect in the field of dye degradation. In addition, a new electrochemical switching ion exchange (ESIX) system is proposed for the treatment of high concentration sodium phenol salt wastewater. A FeHCF coated electrode with large interstitial sites in the rigid open framework was fabricated for the insertion/extraction of Na+ ions from aqueous sodium phenoxide solution by switching the redox state of FeHCF [16].
Table 1
A key direction of future electrochemical oxidation technology research is to develop the combination of electrochemical oxidation and various water treatment processes, overcome the shortcomings of high energy consumption of electrochemical oxidation technology, and achieve the efficient treatment of a large amount of industrial wastewater or dispersed domestic wastewater at a lower cost and in a shorter time.
2.2 Ozonation technology
Ozonation is favored by researchers because of its strong oxidation capacity, rapid degradation efficiency, simple operation, and no secondary pollutants. The ozonation technology can be used for disinfection, improving color in dye wastewater, and solving taste and odor problems (Table 2). There are still many problems with a single ozonation system. On the one hand, the solubility and mass transfer efficiency of ozone in an aqueous solution is very poor, resulting in low practical utilization. On the other hand, the limited oxidation capacity (E0 = 2.07 V) and the selective oxidation of molecular ozone lead to the degradation and mineralization are not ideal. Due to the selective oxidation of ozone and the low utilization efficiency of ozone alone, it is difficult to apply single ozonation technology to the actual high-concentration wastewater treatment project. If the treatment efficiency of ozone can be improved, its application in actual wastewater treatment will be significantly improved.
Table 2
At present, the research focus in the field of ozonation water treatment is to develop efficient multiphase ozonation catalysts. A variety of metals (including Fe, Cu, Pt, Ce, Ru, Mn, etc.) and metal oxides (including Fe2O3, MnO2, MgO, TiO2, Al2O3, CeO2, etc.) coated on porous supports (including activated carbon, alumina, titanium dioxide, silicon dioxide, etc.) have been proposed and studied for ozonation. Song et al. synthesized a magnetic Fe3O4@SiO2@La2O3 nano-catalyst with a core-shell structure to improve the catalytic ozonation efficiency of cinnamyl alcohol. With the help of the catalyst, the degradation rate of cinnamyl alcohol could reach 99.8% at 30 min, and the COD could be removed at 28.5% at 60 min [17]. Its role in development has received more attention due to the inefficiency in the ozonation process and the generation of toxic by-products that limit it. When studying the removal of oxalic acid from water by MnO2/O3, Andreozzi et al. found that increasing the amount of MnO2/O3 catalyst could significantly improve the degradation rate of oxalic acid [18]. Zhu et al. prepared an ordered mesoporous Fe3O4 catalyst by nano casting method for catalytic ozonation degradation of atrazine. The results show that the mesoporous Fe3O4 catalyst has good catalytic activity for the ozonation degradation of atrazine [19]. A composite Mn-Cu-Ce tri-metal oxide supported on γ-Al2O3 (Mn-Cu-Ce/Al2O3) catalyst was prepared by an impregnation calcination method and the synergistic effect of Mn, Cu and Ce oxides greatly enriched the catalytic active center and improved the ozonation performance [20]. Fe-MCM-48 catalyst with three-dimensional cubic pore structure was directly synthesized by hydrothermal method. Compared with single ozonation process, it was found that the mineralization efficiency of the catalyst for diclofenac (DCF) during ozonation was higher [21]. The magnetic Fe3O4@SiO2@CeO2 catalyst, which can be easily separated by magnetic force, was used to catalyze the degradation of ASA in an aqueous solution by ozone. In addition, the Fe3O4@SiO2@CeO2 catalyst has magnetic recyclability and low metal leaching [22]. The catalytic materials of MnOx/γ-Al2O3, CeO2/γ-Al2O3, and MnxCe1-xO2/γ-Al2O3 were synthesized and used in heterogeneous catalytic ozonation of the wastewater containing NFZ. Wherein, MnxCe1-xO2/γ-Al2O3 has excellent catalytic stability, is conducive to recycling, and maintains high activity [23].
2.3 Peroxone technology
As an environmentally friendly, powerful, and common oxidant, H2O2 does not produce toxic residues during decomposition [24]. Therefore, it is commonly used in wastewater treatment. With the addition of H2O2 as the core, a common advanced oxidation process was formed by introducing O3, UV, Fe2+, and other reagents to activate the decomposition process of H2O2 to produce •OH [25]. The peroxone technology involves a series of chain reactions between O3 and H2O2 to generate •OH with strong oxidizing properties. In O3-related advanced oxidation water treatment technology, the consumption and conversion of O3 molecules is a key step in the generation of •OH. The peroxone technology is considered to be a friendly and efficient advanced oxidation technology for wastewater treatment because H2O2 and O3 are green oxidants that can be decomposed into H2O and O2 without producing any secondary by-products [26].
The peroxone technology is a typical advanced oxidation technology, in which additional H2O2 is added on top of ozonation, relying on the reaction of O3 with H2O2 to generate highly reactive radicals •OH with high oxidation capacity. Although the peroxone process can provide a feasible way to treat wastewater, it requires the addition of external H2O2, which is an expensive chemical. Due to the nature of highly concentrated H2O2 solutions, their transportation, storage, and handling are hazardous. These problems greatly limit the practical application of the peroxide process in water treatment [27,28].
3. Characteristics of electro-peroxone technology
3.1 Instrumentation for electro-peroxone technology
The electro-peroxone technology is a novel advanced oxidation process that outperforms ozonation or peroxone technology due to its superiority [27]. This technology, combining ozone and hydrogen peroxide generated electrochemically leads to the production of •OH, which is the strongest oxidizing agent [27,29]. The electro-peroxone technology overcomes the limitation of the conventional peroxone process [30]. The conventional peroxone process requires the addition of H2O2 in the O3 oxidation process. In the electro-peroxone process, H2O2 can be generated on the cathode by O2 in the O3 oxidation process.
A pair of electrodes are installed in the O3 reactor and converted to electro-peroxone equipment. In the electro-peroxone system, the O3 produced by the O3 generator passes through the pipeline to the bottom of the electrochemical device, and the microbubbles are emitted by the aeration head, and the bubbles dissolve the O3 and O2 mixture in the water during the rising process. The O3 system is used to provide a mixture of O2 and O3 to enhance the mass transfer of gaseous O3 to the liquid and the H2O2 aqueous solution generated at the cathode during the reaction, thus synergistically generating stronger •OH.
At present, there is also research on emitting penetrating electro-peroxone ozonation device system. The experimental device is made of plexiglas, mainly composed of an electrochemical workstation, magnetic mixer, calomel reference electrode, penetrating porous membrane electrode as anode, cathode, proton exchange membrane, and so on. In this kind of dual-function system, the cathode as the transmission of the porous composite electrode, effectively breaks traditional electrochemical oxidation fluid mass transfer limit operation system, wastewater in the inside of the membrane pore structure in streaming operation, effectively overcomes the problem of limited mass transfer by streaming operation. The degradation and even mineralization of pollutants can be realized in a very short hydraulic retention time, and a large amount of H2O2 can be produced on the cathode. The constructed dual-function electrolytic system achieves high efficiency in both wastewater oxidation and hydrogen production. This hybrid electrolytic system combines the cathode and anode co-production of H2O2, which has the advantages of wastewater treatment and high value-added chemicals production at the same time (Fig. 1) [31].
Figure 1
A DC power supply is used to apply an electric field to generate H2O2 in situ in electro-peroxone experiments. The generation of H2O2 and •OH is monitored for cathodes, including electrolytic peroxone and electrolysis processes, with oxygen lines connected directly to the bottom of the reaction vessel and ejected for in situ generations of H2O2. The aeration device is introduced from the bottom to produce H2O2 efficiently on the cathode surface, which is carried into the water by a large number of bubbles generated, thus greatly improving the efficiency of •OH production. The O2 in the O3 line is used as input for O3 generation (Fig. 2) [32].
Figure 2
The plexiglas reactor is used for electrochemical ozonation processes and other electrochemical processes and has a size diameter of about 15 cm and a height of about 15 cm. The aeration device consists of an aerator head and a pipe extending into the bottom of the tank, which is a further improvement on the ozonation device and combines electrochemical techniques (Fig. 3) [33].
Figure 3
The reactor size of the electro-peroxone process is larger than conventional advanced oxidation technology. There will be a higher O3 dose which can reduce the reaction time required to effectively degrade O3-refractory pollutants. In an electro-peroxone system, O3 is generated from O2 using an O3 generator. A gas mixture of O2 and O3 from the O3 generator is injected into a reactor. The H2O2 in situ reacts with the sprayed O3 to form a very powerful oxidant •OH, thus achieving the synergistic effect of O3 and H2O2 on the degradation of organic pollutants (Eqs. 3–5) (Fig. 4).
(3) (4) (5) Figure 4
3.2 Advantages of electro-peroxone technology
Given the limitations of currently used oxidation technologies, the development of new advanced oxidation technologies has become a hot research topic in recent years. The advantages of the electro-peroxone technology can be shown through theoretical analysis of the degree of pollutant mineralization, kinetic analysis, and degradation efficiency (Table 3). The electro-peroxone technology can remove most refractory pollutants and typical emerging pollutants [34]. The use of O3/H2O2 and electro-peroxone processes (by adding an external H2O2 reserve or in situ generations of H2O2 through cathodic O2 reduction during ozonation) improved by about 5%-12% and 5%-7%, respectively, compared with conventional ozonation [35]. The electro-peroxone process may provide a simple and effective way to improve the existing ozonation system for pharmaceutical removal from secondary effluents [36]. The degradation of venlafaxine and the removal of total organic carbon (TOC) were greatly enhanced by electro-peroxone treatment due to significant •OH production compared with ozonation and electrolysis alone [37]. The H2O2 generated at the cathode can also effectively quench hypochlorite produced by the oxidation of chloride ions in surface water at the anode. Compared with conventional ozonation, the electro-peroxone process significantly accelerated the removal of refractory micropollutants from surface water [38]. The addition of MnO2 and H2O2 or the H2O2 produced by electrochemistry can promote the conversion of O3 to •OH to varying degrees [39]. The energy consumption in the electro-peroxone water treatment technology can be reduced by the use of circulation system, which can enhance the mass transfer of gasses and pollutants and improve the current efficiency due to the improvement of convection [40]. A flow-through electro-peroxone system consisting of electrolysis and ozonation was also developed for the disinfection of wastewater [41].
Table 3
No chemicals and catalysts are required in the reaction process, and no pH adjustment is needed. It will readily decompose into H2O2 alone, resulting in rapid mineralization of refractory organic pollutants. In general, the reaction in the electro-peroxone system does not leave unwanted by-products (e.g., NH3 and bromate) and catalysts that may require further treatment, separation, and regeneration. In addition, the whole process is electrically driven and therefore easy to automate and control. The H2O2 generated by the cathodic reduction in synergy with ozone can effectively generate •OH, a much more powerful oxidant than O3 and H2O2 alone, resulting in rapid mineralization of refractory organic pollutants, while the process utilizes O2 that would otherwise be wasted to generate H2O2 in situ, thus significantly improving TOC removal efficiency.
4. Mechanism of electro-peroxone technology
In an electro-peroxone system, O2 is passed through a generator to produce O3, and a gas mixture of O2 and O3 is sprayed onto a cathode in an electrochemical reactor that effectively converts O2 to H2O2, for example, a carbon-tetrafluoroethylene (CTPF) cathode, which effectively converts O2 to H2O2. The H2O2 in situ generated subsequently reacts with the injected O3 to produce •OH, an oxidizing agent with high oxidizing power, thus achieving a synergistic effect of O3 and H2O2 on the degradation of organic pollutants. The •OH is a non-selective oxidant that can rapidly oxidize most organic compounds at a diffusion-controlled limited rate.
The electro-peroxone technology is a coupled technology of electrochemical and ozonation technology, during the cathodic reaction, using a carbon cathode and injecting a mixture of O2 with O3 from the O3 generation unit, the main reaction is the reduction of O2 to produce H2O2.
Some reactions may occur during the reduction of O2 to H2O2 on the cathode during the reaction, such as the generation of H2, further reduction of the electrically generated H2O2, and reduction of O3. The table lists the cathodic reactions and the standard reduction potential under alkaline conditions (Table 4). These side reactions affect the generation of H2O2 and the performance of the electro-peroxone process [42]. When the concentration of O3 is too high, the removal rate does not increase. At this point, the mass transfer of O3 is no longer a limiting factor. The current loading capacity reaches saturation, and the reduction generates relatively insufficient H2O2 and inhibits the generation of more •OH. The excess O3 reacts slowly with pollutants and may be reduced to O2 at the cathode, competing with oxygen reduction and further reducing the production of H2O2, so that •OH production decreases and degradation efficiency is affected. When the cathodic O3 reduction current is limited, O3 can be reduced preferentially than O2 at the carbon cathode due to the high potential, which prevents the cathodic O2 reduction to H2O2 and subsequent reactions in the electrochemical-driven peroxone process H2O2 and O3 become •OH [42]. TOC removal in the electro-peroxone process is not limited by the rate of H2O2 generation but is more likely to be limited by the rate of O3 transfer from the gas phase to the liquid phase. However, due to the poor solubility of O3 in water, the rate of transfer of O3 from the gas phase to the liquid phase may eventually limit the rate of •OH generation as the current gradually increases. Side reactions are undesirable in the electro-peroxone process because they impair the production of H2O2 and thus hurt water treatment [43].
Table 4
Table 4. Possible cathodic reactions that may occur during the electro-peroxone process and their standard electrode potentials (E0).a4.1 Selection of electrode materials
In the electro-peroxone process, the generation of H2O2 in situ is essential to achieve the synergistic effect of peroxide on the degradation of pollutants. Therefore, the selection of cathode materials that can efficiently produce H2O2 from oxygen is crucial for the success of the electro-peroxone process. Many carbon electrodes are available for H2O2 production, such as graphite, carbon PTFE, carbon felt, RVC, carbon nanotubes, and graphene electrodes. It showed that pulsed electrochemical oxidation using PTFE-Fe-PbO2 electrodes may be a promising alternative for improving the practicality of electrochemical treatment of dye wastewater [44]. Among the three carbon-based cathodes (carbon-PTFE, carbon felt, and RVC), the carbon-PTFE cathode achieved the highest TOC removal yield, which can mainly be attributed to its highest electrocatalytic activity for H2O2 generation from O2 reduction [45]. The performance of many other carbon-based cathodes (such as plain metal foam electrodes, carbon-coated metal foam electrodes, and sponge electrodes) is still unexplored for the application of electro-peroxone [46]. The Carbon-PTFE electrode has higher H2O2 yield and current efficiency than the general electrode, and the hydroxyl radical yield and current efficiency under alkaline conditions are better than that under acidic conditions. A vast number of studies have demonstrated that although many different carbon-based electrodes can be used to generate H2O2 from O2 reduction, carbon-PTFE is usually the most effective electrode and can produce H2O2 with current efficiency approaching 100%.
Nickel foam usually has good structural characteristics, such as a three-dimensional porous structure, high specific surface area, good electrical conductivity, and low cost. The porous structure and high surface area lead to better mass transfer and active sites for oxygen reduction reactions (Eqs. 6 and 7)
(6) (7) The dissolved oxygen in the pollutant solution is reduced in situ at the cathode to generate H2O2, which is effectively decomposed by O3 in the cathode catalyst layer. The •OH is produced to achieve efficient degradation of pollutants, and the penetration of cathode materials can effectively improve the mass transfer efficiency of pollutant molecules [47].
Based on anodic oxidation and cathodic reduction, the cathode-anode collaboration technology can also be developed, that is, the double-electrode reaction method can be adopted to further improve the current utilization rate, enhance the cathodic reaction, and improve the degradation rate of refractory organic pollutants. The most selected cathode materials are mainly graphite, mesh porous electrode, and Carbon-PTFE gas diffusion electrode. Studies have shown that the Carbon-PTFE gas diffusion electrode has higher H2O2 yield and current efficiency than the graphite electrode [43].
In the research and development of electro-peroxone technology, it is found that changing the carbon-PTFE gas diffusion cathode to a titanium sheet, boron-doped diamond film electrode, and stainless steel cathode in electro-peroxone technology formed another type of ozonation structure treatment technology, which is better than traditional ozonation and electrochemical oxidation technology. Also, the results show that the H2O2 concentration generated in the electrolysis system is notably increased in the presence of TiO2-GAC (Fig. 5) [48]. And the actual current efficiency of cathodic generation of H2O2 is expected to be higher due to the higher hydrogen precipitation overpotential and lower H2O2 decomposition catalytic activity of carbon-based electrodes or autolytic decomposition in the native solution.
Figure 5
The •OH is generated by cathodic O2 reduction using carbon-based electrodes due to their higher hydrogen precipitation overpotential and lower H2O2 decomposition catalytic activity (Eq. 6) or autolytic decomposition in the native solution (Eq. 7) [44,49].
4.2 Effect of current density
The generation of H2O2 is an important factor affecting the treatment effect of the electro-peroxone technology [20]. The current efficiency of H2O2 is different under different currents. The concentration of H2O2 increases with the current, and there is a linear relationship between the concentration of H2O2 and the reaction time [43]. However, the mass transfer process of H2O2 is limited after some time, and the further upgrade of the current has little influence on H2O2. Due to the poor solubility of O3 in water, the rate of transfer of O3 from the gas phase to the liquid phase may eventually limit the rate of •OH production as the current gradually increases [50]. The efficiency of H2O2 generation at the electrode can be verified by Eq. 8 under different current conditions, thus investigating the effect of current density on the reaction in the electro-peroxone system [45]. The rate of H2O2 anodic decomposition increases with the concentration of H2O2 in the solution, and the apparent current efficiency for H2O2 generation decreased slightly as the process proceeds. Compared with the carbon-PTFE cathode, the carbon felt and RVC cathodes generated H2O2 at much lower apparent current efficiencies [51].
(8) The generation of H2O2 in situ from a Carbon-PTFE gas diffusion electrode is a current-limited process. High current density does not significantly improve the removal efficiency, probably because of the increased side reaction and ohmic heat loss at higher current density. Therefore, it is necessary to select the best current density for the experiment.
4.3 Synergistic effects of O2 and O3 gas mixtures
The content of O3 is one of the important factors of the electro-peroxone process. Different O3 levels have different effects on the degradation of the target pollutants in wastewater. O3 usually accounts for only a small fraction of the ozone generator gas effluent, since the ozone generator can only convert a small fraction of the feed O2. In conventional ozonation treatment, O2 (which is the major part of the injected gas) is wasted. The electro-peroxone technology, when the electrochemical oxidation and ozonation technology are simply combined to drive the electro-peroxone reaction to generate a large number of •OH.
In energy, only a small fraction of O2 is converted to O3, and a large fraction of O2 and O3 in the ozone generator is unconverted O2. The gas mixture leaving the ozone generator still contains a large amount of O2. When using pure O2 as the feed gas, the volume ratio of the O2/O3 mixture is usually greater than 90% [52]. After the injection of O3 into the reactor, a little O2 is used to remove contaminants. This wastes large amounts of oxygen feed gas and energy. In the electro-peroxone process, the H2O2 generated by the cathodic reduction in synergy with O3 effectively produces •OH, which are much more powerful oxidant than O3 and H2O2 alone, resulting in rapid mineralization of refractory organic pollutants (Table 5) [40].
Table 5
5. Application of electro-peroxone technology
The electrochemical oxidation technology is widely used in water treatment. The mild processing conditions, under the atmospheric pressure, but it is controlled by many factors, such as the electrode material, electrolyte concentration, voltage, etc., in a relatively high current, easy to produce side effects, in addition, in the process of oxidation of organic compounds produced by free radical polymerization deposition on the electrode surface, which can lead to current efficiency drops, high-performance anode prices more expensive. In order to reduce the problem of electrochemical oxidation degradation process and the problem of ozonation technology, the electro-peroxone technology is currently applied to wastewater. The electro-peroxone technology is a novel advanced oxidation technology.
The higher removal efficiency of NB by electro-peroxone-ACF can be attributed to the synchronous adsorption of NB and O3 on ACF and the remarkable generation of •OH from sparged O3 and electro-catalytic production of H2O2 on ACF (Fig. 6) [53].
Figure 6
It has been shown that in the ozonation of bromine-containing water, the electro-generation of H2O2 can effectively inhibit the formation of bromate and improve the removal of NOM [38]. This result is similar to the process of peroxone, in which H2O2 is added externally. However, H2O2 in situ can successfully overcome the main drawbacks of the conventional peroxide oxidation process. The high chemical cost and the risk associated with the use of an outbound H2O2 solution severely limit its application in practical water treatment. In the study, we used an ACF electrode and driven electro-peroxone process to achieve efficient removal of Cr(Ⅲ) organic complex, while simultaneously recovering Cr from wastewater and enhancing the reduction of intermediate Cr(Ⅵ) (Fig. 7) [54].
Figure 7
In addition, H2O2 can react with many species that may be present in water and wastewater [55]. These reactions can have complex effects on the performance of electro-peroxone technology for water and wastewater treatment. This technology combines the nature of carbon electrode and ozone technology, which can transform the oxygen in the O2/O3 mixture into H2O2 in situ, and then use the Related advanced oxidation techniques combining ozonation technology and electrochemical oxidation technology to produce •OH, so as to degrade the pollutants. Due to the complex role of the water matrix and the interaction between the native and electrode reactions, more research is needed to better understand the reaction mechanisms involved in the electro-peroxone process.
6. Conclusions and perspectives
6.1 Conclusions
The electro-peroxone technology has made some progress in the wastewater treatment process with the advantages of high degradation efficiency, TOC mineralization, the short reaction time required for water treatment, and low energy consumption. Meanwhile, the research mechanism and result of the electro-peroxone technology are introduced from two aspects: cathodic reaction and bulk reaction. The influence of experimental parameters on the wastewater treatment effect is also discussed.
The electro-peroxone technology overcomes some disadvantages and inherent limitations of traditional advanced oxidation technology in sewage treatment, energy saving, and emission reduction. It has improved the performance of wastewater treatment in many aspects and achieved more satisfactory results. In general, the following advantages of the electro-peroxone technology make it a feasible new option for advanced oxidation technology wastewater treatment applications: (1) Simple modifications and upgrades of existing oxidation system equipment. (2) High O3 utilization and low energy consumption. (3) High degradation efficiency mean that •OH is efficiently generated under the synergistic action of O3 and H2O2. (4) Less by-product generation means less secondary pollution, thus protecting the environment. (5) Low risk, that is, it solves the safety problem of transporting H2O2 outward. (6) High pollutant degradation kinetics and energy efficiency.
6.2 Perspectives
So far, although the electro-peroxone technology has shown excellent performance in advanced oxidation technologies, there are still some aspects that need more attention in future research. Future research will focus on the following aspects.
(1) At present, many experimental studies on the wastewater treatment of refractory organic compounds have been carried out and achieved success, providing important information on reaction kinetics and mechanisms, water matrix effects, energy efficiency, etc., but most of them are still in the pilot stage and have not been put into practical application.
(2) Due to the different structures of the reaction equipment, the electro-peroxone technology needs to focus on the complex effects in water and the formation mechanisms and control strategies of reactions in the process, and conduct more detailed pilot evaluations so that it can be put into practical wastewater treatment.
(3) The decomposition of O3 and H2O2 on the cathode into •OH in the electro-peroxone system, which may lead to the disinfection of the process that O3 is both an oxidant and disinfectant in wastewater treatment, improves the oxidation capacity of the process compared to the conventional O3 oxidation system, but the disinfection performance needs to be optimized and evaluated in future studies.
(4) The electro-peroxone technology can generate more •OH and shows an obvious synergistic effect of electrochemical oxidation and ozonation for wastewater treatment, which can be potentially widely used in wastewater treatment.
Declaration of competing interest
The authors declare that they have no known competing financial interests or personal relationships that could have appeared to influence the work reported in this paper.
Acknowledgments
The authors are grateful for the financial support provided by the National Natural Science Foundation of China (No. 21306175), the Zhejiang Provincial Natural Science Foundation of China (No. LGJ18E080001), the Project of Science and Technology Department of Jiashan (Nos. 2020D02 and 2022A23) and Zhejiang Province (No. 2015C03017).
-
-
[1]
R.B. Carneiro, C.M. Mukaeda, C.A. Sabatini, L. Neto, M. Zaiat, J. Environ. Manag. 273 (2020) 111170. doi: 10.1016/j.jenvman.2020.111170
-
[2]
A.R. Ait, J.E. Gaayda, F.E. Titchou, K. Aicha, M. Hamdani, Desalin. Water Treat. 208 (2020) 407–422. doi: 10.5004/dwt.2020.26446
-
[3]
X. Yang, X. Cheng, A.A. Elzatahry, et al., Chin. Chem. Lett. 30 (2019) 324–330. doi: 10.1016/j.cclet.2018.06.026
-
[4]
S. Guo, Q. Wang, C. Luo, et al., Chemosphere 243 (2020) 125390. doi: 10.1016/j.chemosphere.2019.125390
-
[5]
J. Feng, Q. Tao, H. Lan, Y. Xia, Q. Dai, Chemosphere 286 (2022) 131610. doi: 10.1016/j.chemosphere.2021.131610
-
[6]
Q. Dai, L. Wang, K. Wang, et al., Adv. Funct. Mater. 32 (2021) 2109556.
-
[7]
C. Qiu, S. Yuan, X. Li, et al., J. Hazard. Mater. 280 (2014) 644–653. doi: 10.1016/j.jhazmat.2014.09.001
-
[8]
U.V. Gunten, J. Hoigne, Environ. Sci. Technol. 13 (1995) 45–50.
-
[9]
J. Feng, H. Lan, Q. Tao, W. Chen, Q. Dai, Chem. Eng. J. 916 (2022) 116305.
-
[10]
Y. Xia, G. Wang, L. Guo, Q. Dai, X. Ma, Chemosphere 241 (2020) 125010. doi: 10.1016/j.chemosphere.2019.125010
-
[11]
Y. Xia, J. Feng, S. Fan, W. Zhou, Q. Dai, Chemosphere 263 (2021) 128069. doi: 10.1016/j.chemosphere.2020.128069
-
[12]
H. Lan, Q. Tao, N. Ma, et al., Sep. Purif. Technol. 301 (2022) 121856. doi: 10.1016/j.seppur.2022.121856
-
[13]
Z. Pan, H. Xin, S. Xu, et al., Sep. Purif. Technol. 287 (2022) 120600. doi: 10.1016/j.seppur.2022.120600
-
[14]
D. Yu, J. Cui, X. Li, H. Zhang, Y. Pei, Chemosphere 243 (2020) 125438. doi: 10.1016/j.chemosphere.2019.125438
-
[15]
T. Zhang, J. Xu, J. Qian, J. Zhang, J. Mater. Sci. 55 (2020) 13605–13617. doi: 10.1007/s10853-020-04998-5
-
[16]
X. Zhang, J. Niu, X. Hao, et al., Sep. Purif. Technol. 248 (2020) 117125. doi: 10.1016/j.seppur.2020.117125
-
[17]
J. Song, N. Ma, W. Chen, J. Chen, Q. Dai, Sep. Purif. Technol. 282 (2022) 119969. doi: 10.1016/j.seppur.2021.119969
-
[18]
R. Andreozzi, A. Insola, V. Caprio, Appl. Catal. A Gen. 138 (1996) 75–81. doi: 10.1016/0926-860X(95)00247-2
-
[19]
S. Zhu, B. Dong, Y. Yu, et al., Chem. Eng. J. 328 (2017) 527–535. doi: 10.1016/j.cej.2017.07.083
-
[20]
K.H. Zhao, Y.L. Ma, F. Lin, S.Y. Ge, L. Zhu, Environ. Sci. Pollut. Res. 3 (2021) 1–12.
-
[21]
X. Li, W. Chen, Y. Tang, L. Li, Chemosphere 2006 (2018) 615–621.
-
[22]
Q. Dai, J. Wang, J. Yu, Appl. Catal. B 144 (2014) 686–693. doi: 10.1016/j.apcatb.2013.05.072
-
[23]
N. Ma, Y. Ru, M. Weng, et al., Chemosphere 308 (2022) 136192. doi: 10.1016/j.chemosphere.2022.136192
-
[24]
A.D. Bokare, W. Choi, J. Hazard. Mater. 275 (2014) 121–135. doi: 10.1016/j.jhazmat.2014.04.054
-
[25]
S. Li, G. Zhang, W. Zhang, et al., Chem. Eng. J. 326 (2017) 756–764. doi: 10.1016/j.cej.2017.06.037
-
[26]
Y. Liu, J. Jiang, J. Ma, et al., Water Res. 68 (2015) 750–758. doi: 10.1016/j.watres.2014.10.050
-
[27]
S. Yuan, Z. Li, Y. Wang, Electrochem. Commun. 29 (2013) 48–51. doi: 10.1016/j.elecom.2013.01.012
-
[28]
H. Zhang, Q. Ji, L. Lai, G. Yao, B. Lai, Chin. Chem. Lett. 30 (2019) 1129–1132. doi: 10.1016/j.cclet.2019.01.025
-
[29]
M. Ghalebizade, B. Ayati, Chemosphere 235 (2019) 1007–1014. doi: 10.1016/j.chemosphere.2019.06.211
-
[30]
G. Merenyi, J. Lind, S. Naumov, et al., Chem. Eur. J. 16 (2010) 1372–1377. doi: 10.1002/chem.200802539
-
[31]
D. Wu, Y. Shen, G. Lu, Y. Zhou, C. Zhou, Sep. Purif. Technol. 260 (2021) 118202. doi: 10.1016/j.seppur.2020.118202
-
[32]
R. Srinivasan, I.M. Nambi, Chem. Eng. J. 450 (2022) 137618. doi: 10.1016/j.cej.2022.137618
-
[33]
M. Kashani, R. Kiani, A. Hassani, et al., Sep. Purif. Technol. 292 (2022) 121026. doi: 10.1016/j.seppur.2022.121026
-
[34]
Z. Guo, Y. Xie, Y. Wang, et al., Chem. Eng. J. 337 (2018) 733–740. doi: 10.1016/j.cej.2017.11.178
-
[35]
H. Wang, J. Zhan, W. Yao, et al., Water Res. 130 (2018) 127–138. doi: 10.1016/j.watres.2017.11.054
-
[36]
W. Yao, X. Wang, H. Yang, et al., Water Res. 88 (2016) 826–835. doi: 10.1016/j.watres.2015.11.024
-
[37]
X. Li, Y. Wang, J. Zhao, et al., J. Hazard. Mater. 300 (2015) 298–306. doi: 10.1016/j.jhazmat.2015.07.004
-
[38]
W. Yao, J. Fu, H. Yang, G. Yu, Y. Wang, Water Res. 157 (2019) 209–217. doi: 10.1016/j.watres.2019.03.049
-
[39]
Y. Guo, E. Zhao, J. Wang, et al., J. Hazard. Mater. 389 (2020) 121829. doi: 10.1016/j.jhazmat.2019.121829
-
[40]
Y. Zhang, S. Zuo, Y. Zhang, et al., J. Hazard. Mater. 368 (2019) 771–777. doi: 10.1016/j.jhazmat.2019.02.005
-
[41]
Y. Zhang, S. Zuo, Y. Zhang, et al., Chem. Eng. J. 348 (2018) 485–493. doi: 10.1016/j.cej.2018.04.123
-
[42]
Y. Gang, X. Guang, D. Wang, et al., Water Res. 118 (2017) 26–38. doi: 10.1016/j.watres.2017.04.005
-
[43]
Y. Wang, Y. Gang, S. Deng, Chemosphere 208 (2018) 640–654. doi: 10.1016/j.chemosphere.2018.05.095
-
[44]
X. Ma, Y. Yan, Q. Dai, et al., Sep. Purif. Technol. 279 (2021) 119775. doi: 10.1016/j.seppur.2021.119775
-
[45]
H. Munedzimwe, S.V. Rotkin, J. Hazard. Mater. 319 (2016) 61–68. doi: 10.1016/j.jhazmat.2015.12.054
-
[46]
Q. Huang, S. Deng, D. Shan, et al., J. Colloid Interface Sci. 488 (2017) 142–148. doi: 10.1016/j.jcis.2016.11.001
-
[47]
U.V. Gunten, Environ. Sci. Technol. 52 (2018) 5062–5075. doi: 10.1021/acs.est.8b00586
-
[48]
G. Asgari, A. Seid-Mohammadi, A. Rahmani, et al., Chemosphere 266 (2020) 129179.
-
[49]
B. Sljukic, C.E. Banks, R.G. Compton, J. Iran. Chem. Soc. 2 (2005) 1–25. doi: 10.1007/BF03245775
-
[50]
Z. Hui, D. Zhang, J. Zhou, J. Hazard. Mater. 135 (2006) 106–111. doi: 10.1016/j.jhazmat.2005.11.025
-
[51]
E. Brillas, I. Sires, M. turan, Chem. Rev. 109 (2009) 6570–6631. doi: 10.1021/cr900136g
-
[52]
H. Wang, S. Yuan, J. Zhan, et al., Water Res. 80 (2015) 20–29. doi: 10.14355/ijep.2015.04.003
-
[53]
X. Zhang, Y. Zhou, C. Zhao, et al., Chem. Eng. J. 304 (2016) 129–133. doi: 10.1016/j.cej.2016.06.079
-
[54]
C. Chen, P. Liu, Y. Li, et al., Water Res. 218 (2022) 118502. doi: 10.1016/j.watres.2022.118502
-
[55]
Z. Lin, W. Yao, Y. Wang, et al., Water Res. 88 (2016) 691–702. doi: 10.1016/j.watres.2015.11.005
-
[1]
-
Table 1. Status of electrochemical oxidation treatment of refractory wastewater.
Table 2. Status of treatment of refractory wastewater by ozonation.
Table 3. Status of treatment of refractory wastewater by ozonation.
Table 4. Possible cathodic reactions that may occur during the electro-peroxone process and their standard electrode potentials (E0).a
Table 5. Reaction during the electro-peroxone process.
-

计量
- PDF下载量: 8
- 文章访问数: 532
- HTML全文浏览量: 64