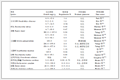

NH2-Ga-MIL-53催化剂对Strecker反应的底物选择性
-
关键词:
- 金属有机骨架配合物
- / 镓
- / 非均相催化
- / Strecker反应
- / 底物选择性
English
Substrate-Selectivity of Strecker Reaction Based on Amino-Functionalized Ga-MIL-53 Catalyst
-
Key words:
- metal-organic frameworks
- / gallium
- / heterogeneous catalysis
- / Strecker reaction
- / substrate-selectivity
-
0. Introduction
Strecker-type reactions, involving nucleophilic addition of cyanide sources to aldimine, have become one of the most versatile and efficacious approaches in the yielding of α-aminonitriles on lab and technical scale[1-5]. To enhance the conversion efficiency of Strecker-type reactions, several heterogeneous catalysts such as metal complexes[6], metal-salen complexes[7-8] and metal oxides[9] have been extensively investigated over the past few years, owing to the advantages of catalysts recycle as well as product separation. For instance, a molybdenum Schiff base complex supported on MNPs (magnetic nanoparticles) has been utilized as an efficient and easily recyclable catalyst for the syntheses of α-aminonitrile derivatives[6]. Most recently, Gao and Yang et al.[9] applied strong Lewis base Ga4B2O9 with GaO connectivity enhanced basicity as well as Lewis acidic sites of five-coordinated Ga3+ as an efficient catalyst to facilitate Strecker-type reactions. Now the Strecker reactions have gained extensive research interests during the recent years, becoming the hot topic in the field of catalysis.
Metal organic frameworks (MOFs)[10-15] have attracted widespread attention in the promotion of various organic reactions, especially for Strecker reaction, showing several essential advantages[16-21]. On the one hand, by appropriately selecting Lewis acid centers of metal-containing secondary building units[22-23] and organic linkers with potential Lewis base sites[24-26], MOFs demonstrated impressive attractions to achieve high performances for Lewis acid-base bifunctional activations[27-28]. In 2014, Indium(Ⅲ)-based MOFs were first reported as recyclable heterogeneous Lewis acid-base bifunctional catalysts for Strecker reactions, while cyanide source of trimethylsilyl cyanide (TMSCN) can be activated by Lewis basic sites of exposed ether groups, which has been confirmed by 29Si NMR[29]. On the other hand, as other heterogeneous catalysts, the heterogeneous nature of MOFs can readily promote catalyst recycling and product separation[27, 30-31]. For instance, Monge and Gándara et al. have developed a new highly porous and heterogeneous Lewis acid catalyst of Indium(Ⅲ)-based MOF to facilitate Strecker-type reactions, which can be recycled for 10 runs without significant loss of yield[32].
To our knowledge, in the case of Strecker reactions, the detailed substrate-selectivity patterns for substrates with complex combination between electron-withdrawing and electron-donating substituents have not been summarized in previous reported papers. Herein, we employed a series of N-Ph aldimines as substrates to study these complicated substrate-selectivity regulars with respect to Strecker reactions. Meanwhile, the present work focuses on the catalytic research of amino-functionalized Ga-MIL-53 (NH2-Ga-MIL-53) material in Strecker reactions after considering the above-mentioned advantages of the catalysts of MOFs. As a result, NH2-Ga-MIL-53 exhibited excellent catalytic performance in Strecker reactions as well as significant catalytic universality for numerous substrates of aldimines. Most importantly, the intricate influences on Strecker reaction with various substrates were firstly discussed in this work. Noteworthy, as a heterogeneous catalyst, NH2-Ga-MIL-53 can be recycled for 9 runs with no obvious loss of conversion efficiencies. Furthermore, the influence of Lewis base amino moieties[33-34] on conversion efficiencies, along with the effect of the structure of NH2-Ga-MIL-53 framework on the catalytic performance, have been explored during the present work.
1. Experimental
1.1 Materials and instrumentation
All solvents and reagents adopted in this work were of reagent grade and utilized with no further purification. X-ray diffraction (XRD) was performed by Rigaku D/max-2550 diffractometer with copper anode and graphite monochoromator to select Cu Kα1 radiation (λ=0.154 18 nm with an operating voltage of 40.0 kV and a beam current of 30.0 mA) at room temperature, and XRD data were collected in a 2θ range of 4°~40° with a scanning rate of 6°·min-1. Thermogravimetry (TGA) was performed with a Perkin-Elmer TGA 7 Instruments equipment under air atmosphere with a heating rate of 10 ℃·min-1 (the temperature range was from 16 to 800 ℃). IR spectra were collected on a Nicolet FT-IR 20SXC spectrometer from KBr pellets in a range of 4 000~400 cm-1, while pure KBr peaks were excluded as background, and IR spectra was processed with data turn-up and smoothing. N2 adsorption-desorption isotherms were recorded on an ASAP 2020 surface area and pore volume automatic analysis instrument. 1H NMR spectra was collected by a Bruker Avance 400 equipment with a test frequency of 400 MHz.
1.2 Catalyst synthesis
1.2.1 Synthesis of NH2-Ga-MIL-53
The synthesis of NH2-Ga-MIL-53 was adapted from that of amino-functionalized Al-MIL-53[35-36]. At first, 1.05 mmol Ga(NO3)3·6H2O was dissolved into 7.5 mL N, N-dimethyl-formamide (DMF), while 1.56 mmol 2-aminoterephthalic acid (ATA) was added into another 7.5 mL DMF. These two DMF solutions were mixed together until both Ga(NO3)3·6H2O and ATA were dissolved completely. Hereafter, the mixture was put into a 23 mL Teflon-lined autoclave, and heated under autogenous pressure at 130 ℃ for 3 days. The gel product was separated by centrifugation, washed with acetone and methanol respectively, and refluxed by methanol overnight. Finally, the product was collected by centrifugation and dried under vacuum oven at 110 ℃ for 24 h, then pale-yellow powder was collected.
1.2.2 Synthesis of Ga-MIL-53
Ga-MIL-53 was synthesized by a similar procedure as NH2-Ga-MIL-53, except that ATA was replaced by terephthalic acid. Ultimately, white powder was collected after dried under vacuum oven at 110 ℃ for 24 h.
1.3 General procedure for Strecker reactions
In a typical batch experiment, 0.14 mmol aldimine was dissolved into 2.4 mL CDCl3, followed by 0.04 mmol catalyst (NH2-Ga-MIL-53, Ga-MIL-53, Ga(NO3)3·6H2O or ATA) and 0.35 mmol TMSCN, respectively. The mixture was continuously stirred in a vial, and the conversion efficiencies were recorded by 1H NMR spectra at certain discrete time-points (the time to adding TMSCN was set as the initial point).
1.4 Catalyst recycling
After 99% conversion efficiency aldimines was achieved for each Strecker reaction, the mixture was centrifuged and washed with chloroform for 3 cycles to get the purified precipitates of catalyst. The final precipitates were dried under vacuum oven at 110 ℃ for 24 h.
2. Results and discussion
2.1 Characterizations
All synthetic MIL-53 catalysts were characterized by XRD (Fig.S1), N2 adsorption-desorption analysis (Fig.S2), TGA (Fig.S3) and IR spectra (Fig.S4).
XRD patterns for NH2-Ga-MIL-53 and Ga-MIL-53 are displayed in Fig.S1. As we known, MIL-53 MOFs displayed very large breathing effect with large pore (lp) form and narrow pore (np) form mutual trans-formation[37-39]. It is noted that XRD patterns of NH2-Ga-MIL-53 show np form (CCDC number of Al-MIL-53 with np form: 220477) (Fig.S1a), while XRD pattern of Ga-MIL-53 can be corresponded to opened lp form (CCDC number of Al-MIL-53 with lp form: 220475)[40-41] (Fig.S1b).
Nitrogen adsorption-desorption isotherms at 77 K used for BET (Brunauer-Emmett-Teller) surface area and pore diameter estimations are depicted in Fig.S2 and Table 1. NH2-Ga-MIL-53 performs a Type Ⅲ isotherm characteristic with the BET surface area and pore diameter of 26 m2·g-1 and 0.67 nm, respectively. Ga-MIL-53 exhibited a typical Type Ⅰ isotherm characteristics of microporous materials, while the BET surface area and pore diameter were 1 267 m2·g-1 and 0.61 nm, respectively.
Table 1
Compound BET surface area/(m2·g-1) Langmuir surface area/(m2·g-1) Pore volume/(cm3·g-1) Pore diameter/nm NH2-Ga-MIL-53 26 36 0.058 6.7 Ga-MIL-53 1 267 1 667 0.644 6.1 Evidence for the successful preparation of NH2-Ga-MIL-53 was demonstrated by the IR analyses. As shown in Fig.S4a, the peaks at 3 498 and 3 387 cm-1 were ascribed to the stretching vibration band of N-H in NH2, and the peak at 774 cm-1 was assigned to the bending vibration band of N-H, all results evidently certifying the introducing of amino groups[41].
2.2 Catalytic activity of NH2-Ga-MIL-53 in Strecker reactions
2.2.1 Catalyst activity and universality with various substrates
Initially, experiments were performed with CDCl3 at room temperature to optimize the reaction conditions for the Strecker reaction using benzaldimines as subs-trates, while optimized experimental procedure was illustrated in experimental section. Furthermore, with the purpose of avoiding the use of highly toxic cyanide derivatives, environmentally-benign TMSCN was utilized in our work[6, 42]. As a result, NH2-Ga-MIL-53 performed excellent catalytic performance as well as significant universality for various substrates, giving high conversion efficiencies with a few aldimines (Table 2, entries 1~14). For instance, with the substrate of N-Ph benzaldimine, 85% conversion efficiency was achieved within 11.5 h incubation (Table 2, entry 1), compared with only 2% conversion efficiency at 31.5 h for the substrate with control groups (Table 2, entry 19).
Table 2
Entry R PG Time/h Conversion efficiencya/% 1 Ph Ph 11.5 85 31.5 99 2 Ph 4-MeOPh 11 93 3 2-MeOPh Ph 11 96 4 3-MeOPh Ph 11 91 5 4-MeOPh Ph 11 91 6 4-MeOPh 4-MeOPh 11 93 7 Ph 4-CF3Ph 31.5 81 8 2-CF3Ph Ph 31.5 88 9 3-CF3Ph Ph 31.5 96 10 4-CF3Ph Ph 31.5 84 11 4-CF3Ph 4-CF3Ph 35 29 12 4-CF3Ph 4-MeOPh 31.5 95 13 4-MeOPh 4-CF3Ph 32.5 89 14 4-PhPh Ph 11.5 89 15 4-NO2Ph Ph 11.5 2 16b Ph Ph 23.5 85 17c Ph Ph 0.5 100d 18e Ph Ph 48.5 41d 19f Ph Ph 31.5 2 a Conversion efficiency was confirmed by 1H NMR; b Ga-MIL-53 catalyst was used instead; c Ga(NO3)3·6H2O catalyst was used instead; d Unexpected side reactions procceed largely; e ATA catalyst was used instead; f No catalyst was added Hereafter, on the basis of substrates with PGs (PGs=protecting groups) of Ph, the effect of electron-withdrawing and electron-donating substituted groups of aldimines on the reactivity was preliminarily investigated. In the case of 4-substituted aldimines, substrates with electron-withdrawing groups (i.e., CF3, NO2) gave much slower reaction rates compared to that of N-Ph benzaldimine (Table 2, entries 1, 10 and 15), and substrates with electron-donating groups (i.e., MeO, Ph) afford higher conversion efficiencies in comparison with that of N-Ph benzaldimine (Table 2, entries 1, 5 and 14). Furthermore, as achieving conversion efficiencies of 99% among substrates of 3-substituted aldimines, the substrate with R group of 3-MeOPh required merely 24.5 h while aldimine with R group of 3-CF3Ph demanded up to 48.5 h, and aldimine with R group of Ph required 31.5 h under identical conditions (Fig.S5a and Table S1, entries 1, 4 and 9). In conclusion, the electron-withdrawing groups of aldimines significantly retard the Strecker-type reaction rate, while the electron-donating groups of aldimines slightly advance the reactivity of substrates. It can be deduced that the electron-withdrawing substituted groups can weaken the electrophilic Ga coordination with nucleophilic nitrogen atoms of aldimines via lowering the nucleophilic nature of aldimines according to the inductive effect of electron-withdrawing substituents[43], hindering the subsequent reactions for synthesis of α-aminonitriles.
Furthermore, it should be noted that reaction rates of aldimines depend on the position of substituents and decrease in the following order: R group of 2-MeOPh > PG of 4-MeOPh≈R group of 3-MeOPh≈R group of 4-MeOPh (hereafter; both R groups and PGs are Ph unless specially mentioned) (Fig.S5b and Table 2, entries 2, 3, 4 and 5). In addition, with further increase of the number of MeO substitutions, this reaction rate no longer increased (Fig.S5b and Table 2, entry 6). Apart from MeO substituents, one can see from Fig.S5c that reaction rates of aldimines also depended on the position of CF3 substituents and decreased in the following order: R group of 3-CF3Ph > R group of 2-CF3Ph > R group of 4-CF3Ph > PG of 4-CF3Ph (Table 2, entries 7, 8, 9 and 10). With further increase of the number of CF3 substitutions, it performs the worst catalytic effect among all the substrates (Fig.S5c and Table 2, entry 11). It can be concluded: (1) the ortho-substituted and PG-substituted aldimines perform the most obvious promotion and suppression effects on the reaction for MeO and CF3 substituents, respectively; (2) the multiple MeO groups on substrates facilating the reaction is rather limited, while the multiple CF3 substituents of the substrates obstructing the reaction is illimited.
To investigate the synergy effect between MeO groups and CF3 groups, two substrates of aldimines were selected with PGs of 4-MeOPh and R group of 4-CF3Ph as well as PGs of 4-CF3Ph and R group of 4-MeOPh, respectively. However, both of them showed lower Strecker-type reactivities compared with the aldimine with both R group and PG of Ph (Fig.S5d and Table 2, entries 1, 12 and 13). Herein, we draw the conclusion that CF3 groups performed stronger electron-withdrawing effect than the electron-donating effect of MeO groups, hence reducing the reactivities of these substrates.
2.2.2 Heterogeneity and reusability of catalyst
The heterogeneity and reusability are important properties in the catalytic reactions, which largely reduce the cost and guarantee the high efficiency of product formation[44-46]. For the leaching test, the removal of NH2-Ga-MIL-53 from the system by centrifugation at 2 h can completely terminate the reaction (Fig.S6). It can be concluded that no homogeneous catalyst species existed in the reaction solution[47]. Furthermore, the repeated catalytic experiment of NH2-Ga-MIL-53 was devised. As shown in Fig. 1, NH2-Ga-MIL-53 was easily recovered by centrifugal separation and recycled for 9 runs without significant loss of conversion efficiency at 31.5 h. In addition, this stability of the heterogeneous NH2-Ga-MIL-53 catalyst was revealed by XRD, and no obvious collapse in the structure of np forms was observed after 9-time recycling (Fig.S1c), implying the reusability and structure stability of NH2-Ga-MIL-53 as a catalyst.
Figure 1
2.2.3 Effect of amino groups on the catalyst
To explore the role of Lewis base sites (amino groups) of NH2-Ga-MIL-53 in the Strecker-type reac-tions, Ga-MIL-53 catalyst was used instead. It is noted that NH2-Ga-MIL-53 with np form showed a much smaller BET surface area compared to Ga-MIL-53 with lp form, blocking the reaction because of the limited contact area. However, NH2-Ga-MIL-53 exhibited a better catalytic performance in Strecker-type reaction than Ga-MIL-53 conversely (Fig.S7). Only 11.5 h was required to achieve 85% conversion efficiency for the NH2-Ga-MIL-53 catalyst (Table 2, entry 1), while the conversion efficiency of substrate reached 85% up to 23.5 h in the presence of Ga-MIL-53 (Table 2, entry 16). Therefore, we get the conclusion that the introducing of the Lewis base sites of amino groups for Ga-MIL-53 can readily improve the catalytic performance effectively on the basis of the Lewis acid sites (trivalent Ga cations). This result could be explained as the classic Lewis acid-base synergistic catalytic mechanism[29, 48-49].
2.2.4 Effect of the framework structure of the catalyst
Herein, the influence of the catalyst framework on Strecker reaction was investigated. Firstly, Ga(NO3)3 ·6H2O was used as the catalyst to promote the reaction instead of NH2-Ga-MIL-53. It is observed that this reaction could be finished within 0.5 h, however, the unexpected side reactions procceed largely (Table 2, entry 17). In comparison, NH2-Ga-MIL-53 gave no side reaction but a longer reaction time to achieve high conversion efficiency (Table 2, entry 1). Hereafter, the possible mechanisms were analyzed. On the one hand, the high catalytic activity of Ga(NO3)3·6H2O is caused by the strong Lewis acid metal center, owing to the induction effect of electron-withdrawing nitrate anions[50-53]. On the other hand, because of the lack of pore structure, Ga(NO3)3·6H2O is unable to immobilize the substrate at the catalytic sites, leading to unexpected side reactions. In conclusion, the pore structure of NH2-Ga-MIL-53 framework is essential in enhancing the reaction selectivity while gaining single product[16, 31].
Furthermore, ATA was adopted as catalyst on the Strecker reaction. It affords low conversion efficiency with a much longer reaction time as well as unexpected side products with the catalyst of ATA (Table 2, entry 18). The absence of Lewis acid metal sites for ATA might result in the low conversion efficiency. In addition, this result could further suggest that there is no pore structure for ATA to immobilize the substrate at the reaction sites, leading to poor reaction selectivity and unexpected side reactions[16, 31].
3. Conclusions
In summary, the catalytic activity of NH2-Ga-MIL-53 has been assessed in Strecker reaction, which is found to be a highly reactive catalyst with essential catalytic universality for extensive substrates. Furthermore, the substrate-selectivity patterns for various substrates of N-Ph aldimines were concluded: (1) the electronic effect of substituents primarily influences the reaction rate, while the electron-donating substituents (i.e., MeO, Ph) and electron-withdrawing substituents (i.e., CF3, NO2) exhibit promotion and suppression effects for the reaction, respectively; (2) the substituted position with respect to substituents secondarily impacts the reaction rate, while the ortho-substituted MeO performs the strongest promotion effect on the reaction. As a heterogeneous catalyst, it is noteworthy that NH2-Ga-MIL-53 has a significant structural stability that allows its use in Strecker-type reactions for 9 cycles without obvious loss of activity, being easily recovered after each cycle. Comparing the catalytic effects between NH2-Ga-MIL-53 and Ga-MIL-53, it is illustrated that the amino groups on NH2-Ga-MIL-53 can be served as Lewis base sites, promoting the Strecker reaction via Lewis acid-base synergistic catalytic process. Ultimately, by contrasting the catalytic performances among NH2-Ga-MIL-53, Ga(NO3)3·6H2O and ATA, it is indicated that the pore structure of NH2-Ga-MIL-53 framework plays a major role in improving the reaction selectivity while ensuring the product purity. We expect the present work can provide an idea of developing a catalyst with outstanding reusability, structural stability and remarkable universality and selectivity for different substrates in Strecker reactions to increase the economic effectiveness in industrial application.
Supporting information is available at http://www.wjhxxb.cn
-
-
[1]
Strecker A. Ann. Chem. Pharm., 1850, 75:27-45 doi: 10.1002/jlac.18500750103
-
[2]
Takamatsu N, Aiba S, Yamada T, et al. Chem. Eur. J., 2018, 24:1304-1310 doi: 10.1002/chem.201704033
-
[3]
Ishitani H, Komiyama S, Kobayashi S. Angew. Chem. Int. Ed., 1998, 37:3186-3188 doi: 10.1002/(SICI)1521-3773(19981204)37:22<3186::AID-ANIE3186>3.0.CO;2-E
-
[4]
Iyer M S, Gigstad K M, Namdev N D, et al. J. Am. Chem. Soc., 1996, 118:4910-4911 doi: 10.1021/ja952686e
-
[5]
Wang W L, Zhang S Y, Hu S X, et al. Appl. Catal. A, 2017, 542:240-251 doi: 10.1016/j.apcata.2017.05.030
-
[6]
Rakhtshah J, Salehzadeh S. Res. Chem. Intermed., 2017, 43:6973-6991 doi: 10.1007/s11164-017-3031-3
-
[7]
Saravanan S, Khan N U, Jakhar A, et al. RSC Adv., 2015, 5:99951-99958 doi: 10.1039/C5RA18914D
-
[8]
Hou Y L, Sun R W, Zhou X P, et al. Chem. Commun., 2014, 50:2295-2297 doi: 10.1039/C3CC47996J
-
[9]
Hu S X, Wang W L, Yue M F, et al. ACS Appl. Mater. Interface, 2018, 10:15895-15904 doi: 10.1021/acsami.8b04144
-
[10]
Van Vleet M J, Weng T T, Li X Y, et al. Chem. Rev., 2018, 118:3681-3721 doi: 10.1021/acs.chemrev.7b00582
-
[11]
Cheetham A K, Kieslich G, Yeung H H. Acc. Chem. Res., 2018, 51:659-667 doi: 10.1021/acs.accounts.7b00497
-
[12]
Schoedel A, Li M, Li D, et al. Chem. Rev., 2016, 116:12466-12535 doi: 10.1021/acs.chemrev.6b00346
-
[13]
Furukawa H, Cordova K E, O'Keeffe M, et al. Science, 2013, 341:974-986
-
[14]
Kuppler R J, Timmons D J, Fang Q R, et al. Coord. Chem. Rev., 2009, 253:3042-3066 doi: 10.1016/j.ccr.2009.05.019
-
[15]
刘同飞, 崔广华, 焦翠欢, 等.无机化学学报, 2011, 27(7):1417-1422 http://www.wjhxxb.cn/wjhxxbcn/ch/reader/view_abstract.aspx?file_no=20110734&flag=1LIU Tong-Fei, CUI Guang-Hua, JIAO Cui-Huan, et al. Chinese J. Inorg. Chem., 2011, 27(7):1417-1422 http://www.wjhxxb.cn/wjhxxbcn/ch/reader/view_abstract.aspx?file_no=20110734&flag=1
-
[16]
Liu J W, Chen L F, Cui H, et al. Chem. Soc. Rev., 2014, 43:6011-6061 doi: 10.1039/C4CS00094C
-
[17]
Dhakshinamoorthy A, Asiri A M, Alvaro M, et al. Green Chem., 2018, 20:86-107 doi: 10.1039/C7GC02260C
-
[18]
Vellingiri K, Philip L, Kim K H. Coord. Chem. Rev., 2017, 353:159-179 doi: 10.1016/j.ccr.2017.10.010
-
[19]
Qin J S, Yuan S, Lollar C, et al. Chem. Commun., 2018, 54:4231-4249 doi: 10.1039/C7CC09173G
-
[20]
Verma A, Tomar K, Bharadwaj P K. Inorg. Chem., 2017, 56:13629-13633 doi: 10.1021/acs.inorgchem.7b01915
-
[21]
Gupta V, Mandal S K. Inorg. Chem., 2019, 58:3219-3226 doi: 10.1021/acs.inorgchem.8b03307
-
[22]
Horike S, Dinca M, Tamaki K, et al. J. Am. Chem. Soc., 2008, 130:5854-5855 doi: 10.1021/ja800669j
-
[23]
戴田霖, 张艳梅, 储刚, 等.无机化学学报, 2016, 32(4):609-616 http://www.wjhxxb.cn/wjhxxbcn/ch/reader/view_abstract.aspx?file_no=20160408&flag=1DAI Tian-Lin, ZHANG Yan-Mei, CHU Gang, et al. Chinese J. Inorg. Chem., 2016, 32(4):609-616 http://www.wjhxxb.cn/wjhxxbcn/ch/reader/view_abstract.aspx?file_no=20160408&flag=1
-
[24]
Zhao S X. J. Mol. Struct., 2018, 1167:11-15 doi: 10.1016/j.molstruc.2018.04.078
-
[25]
Song L L, Chen C, Chen X B, et al. Chem. Res. Chin. Univ., 2016, 32:838-842 doi: 10.1007/s40242-016-6076-8
-
[26]
Lin Y C, Kong C L, Chen L. RSC Adv., 2016, 6:32598-32614 doi: 10.1039/C6RA01536K
-
[27]
Kang Y S, Lu Y, Chen K, et al. Coord. Chem. Rev., 2019, 378:262-280 doi: 10.1016/j.ccr.2018.02.009
-
[28]
Huang Y B, Liang J, Wang X S, et al. Chem. Soc. Rev., 2017, 46:126-157 doi: 10.1039/C6CS00250A
-
[29]
Xia J, Xu J N, Fan Y, et al. Inorg. Chem., 2014, 53:10024-10026 doi: 10.1021/ic501492c
-
[30]
Gupta M, De D, Tomar K, et al. Inorg. Chem., 2017, 56:14605-14611 doi: 10.1021/acs.inorgchem.7b02443
-
[31]
Lee J Y, Farha O K, Roberts J, et al. Chem. Soc. Rev., 2009, 38:1450-1459 doi: 10.1039/b807080f
-
[32]
Reinares-Fisac D, Aguirre-Diaz L M, Iglesias M, et al. J. Am. Chem. Soc., 2016, 138:9089-9092 doi: 10.1021/jacs.6b05706
-
[33]
Wang Z X, Ying A G, Fan Z L, et al. ACS Catal., 2017, 7:3676-3680 doi: 10.1021/acscatal.7b00626
-
[34]
Zakharova M V, Masoumifard N, Hu Y M, et al. ACS Appl. Mater. Interface, 2018, 10:13199-13210 doi: 10.1021/acsami.8b00640
-
[35]
Gascon J, Aktay U, Hernandezalonso M D, et al. J. Catal., 2009, 261:75-87 doi: 10.1016/j.jcat.2008.11.010
-
[36]
Couck S, Denayer J F, Baron G V, et al. J. Am. Chem. Soc., 2009, 131:6326-6327 doi: 10.1021/ja900555r
-
[37]
Kim J Y, Zhang L D, Balderas-Xicohtencatl R, et al. J. Am. Chem. Soc., 2017, 139:17743-17746 doi: 10.1021/jacs.7b10323
-
[38]
Liu D D, Yan L T, Li L J, et al. CrystEngComm, 2018, 20:2102-2111 doi: 10.1039/C8CE00050F
-
[39]
Chen L J, Mowat J P, Fairen-Jimenez D, et al. J. Am. Chem. Soc., 2013, 135:15763-15773 doi: 10.1021/ja403453g
-
[40]
Loiseau T, Serre C, Huguenard C, et al. Chem. Eur. J., 2004, 10:1373-1382 doi: 10.1002/chem.200305413
-
[41]
Martínez F, Orcajo G, Briones D, et al. Microporous Mesoporous Mater., 2017, 246:43-50 doi: 10.1016/j.micromeso.2017.03.011
-
[42]
Martínez R, Ramón D J, Yus M. Tetrahedron Lett., 2005, 46:8471-8474 doi: 10.1016/j.tetlet.2005.10.020
-
[43]
Ohmori O, Fujita M. Chem. Commun., 2004, 4:1586-1587 doi: 10.1039/b406114b
-
[44]
Chughtai A H, Ahmad N, Younus H A, et al. Chem. Soc. Rev., 2015, 44:6804-6849 doi: 10.1039/C4CS00395K
-
[45]
Dhakshinamoorthy A, Garcia H. Chem. Soc. Rev., 2014, 43:5750-5765 doi: 10.1039/C3CS60442J
-
[46]
陈秀莹, 谢慧琳, 胡文斌, 等.无机化学学报, 2018, 34(5):933-941 http://www.wjhxxb.cn/wjhxxbcn/ch/reader/view_abstract.aspx?file_no=20180515&flag=1CHEN Xiu-Ying, XIE Hui-Lin, HU Wen-Bin, et al. Chinese J. Inorg. Chem., 2018, 34(5):933-941 http://www.wjhxxb.cn/wjhxxbcn/ch/reader/view_abstract.aspx?file_no=20180515&flag=1
-
[47]
Xia J, Zheng J F, Xu J N, et al. Inorg. Chim. Acta, 2014, 411:35-39 doi: 10.1016/j.ica.2013.11.014
-
[48]
Reid J P, Simon L, Goodman J M. Acc. Chem. Res., 2016, 49:1029-1041 doi: 10.1021/acs.accounts.6b00052
-
[49]
Simon L, Goodman J M. J. Am. Chem. Soc., 2009, 131:4070-4077 doi: 10.1021/ja808715j
-
[50]
Wang Y, Ma J H, Liang D, et al. J. Mater. Sci., 2009, 44:6736-6740 doi: 10.1007/s10853-009-3603-8
-
[51]
Chen J X, Wu H Y, Zheng Z G, et al. Tetrahedron Lett., 2006, 47:5383-5387 doi: 10.1016/j.tetlet.2006.05.085
-
[52]
Ma Y, Qian C T, Wang L M, et al. J. Org. Chem., 2000, 65:3864-3868 doi: 10.1021/jo9919052
-
[53]
Ahrem L, Wolf J, Scholz G, et al. Catal. Sci. Technol., 2018, 8:1404-1413 doi: 10.1039/C7CY02257C
-
[1]
-
Table 1. Parameters of pore collected by N2 adsorption analysis
Compound BET surface area/(m2·g-1) Langmuir surface area/(m2·g-1) Pore volume/(cm3·g-1) Pore diameter/nm NH2-Ga-MIL-53 26 36 0.058 6.7 Ga-MIL-53 1 267 1 667 0.644 6.1 Table 2. Strecker-type reactions for NH2-Ga-MIL-53 catalyst
Entry R PG Time/h Conversion efficiencya/% 1 Ph Ph 11.5 85 31.5 99 2 Ph 4-MeOPh 11 93 3 2-MeOPh Ph 11 96 4 3-MeOPh Ph 11 91 5 4-MeOPh Ph 11 91 6 4-MeOPh 4-MeOPh 11 93 7 Ph 4-CF3Ph 31.5 81 8 2-CF3Ph Ph 31.5 88 9 3-CF3Ph Ph 31.5 96 10 4-CF3Ph Ph 31.5 84 11 4-CF3Ph 4-CF3Ph 35 29 12 4-CF3Ph 4-MeOPh 31.5 95 13 4-MeOPh 4-CF3Ph 32.5 89 14 4-PhPh Ph 11.5 89 15 4-NO2Ph Ph 11.5 2 16b Ph Ph 23.5 85 17c Ph Ph 0.5 100d 18e Ph Ph 48.5 41d 19f Ph Ph 31.5 2 a Conversion efficiency was confirmed by 1H NMR; b Ga-MIL-53 catalyst was used instead; c Ga(NO3)3·6H2O catalyst was used instead; d Unexpected side reactions procceed largely; e ATA catalyst was used instead; f No catalyst was added -

计量
- PDF下载量: 3
- 文章访问数: 617
- HTML全文浏览量: 45