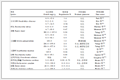

Crystal Structure, Fe3+ Luminescence Sensing and Color Tuning of 2D Lanthanide-metal-organic Frameworks Constructed from Tricarboxylic Acid Ligand
English
Crystal Structure, Fe3+ Luminescence Sensing and Color Tuning of 2D Lanthanide-metal-organic Frameworks Constructed from Tricarboxylic Acid Ligand
-
Key words:
- lanthanide metal-organic frameworks
- / sensing
- / luminescence
- / Fe3+
- / color tuning
-
1. INTRODUCTION
Iron(Ⅲ), as an indispensable trace elements of our life, plays an important role in a number of physiological processes such as DNA synthesis, hemoglobin formation, oxygen transport and storage, and coordination of brain functions[1-5]. However, the presence of a large amount of iron(Ⅲ) is harmful to humans[6, 7], causing damages to heart, liver, and other vital organs through the blood circulation, and hence in turn affects the absorption of other elements[8, 9]. Therefore, detection of iron(Ⅲ) in human body is getting greater attention where luminescent sensing has been marked as an efficient tool to achieve this task based on simplified and convenient operation[10-12].
Recently, metal-organic frameworks (MOFs) as new materials have been widely applied in numerous areas, including gas storage and separation, catalysis, optoelec-tronics, energy storage and conversion, photocatalysis and luminescence and sensing[13-24]. As a promising subfamily of MOFs, lanthanide based MOFs (Ln-MOFs) have attracted great attention for their significant luminescent properties because of the intrinsic features of lanthanide elements. Ln-MOFs have unique advantages and properties due to the 4f electron configuration of the lanthanide metal, such as strong absorption and inherent luminescence band of lanthanides, large Stokes shift, pure color, and relatively long luminescence lifetime, which make them receive more attention in luminescence sensing[25-33]. Furthermore, the fluorescent properties of Ln-MOFs can be further modulated by both analyte-metal and analyte-ligand interactions since the unique luminescence mechanism involves ligand-to-metal energy transfer (LMET)[34-36]. In particular, Eu3+ and Tb3+ based complexes have great significance for the luminescent studies because they can emit strong characteristic red or green light when excited by energy transfer from the ligand to the lanthanide ion via UV radiation. Moreover, mixed-Ln-MOFs can be obtained through integrating Eu3+ and Tb3+ into a single crystal of Ln-MOFs, which can effectively combine dual-luminescent centers to generate multicolored emission materials. In general, the changes in the emission color can be effectively modulated by changing the mixing ratios of Eu3+: Tb3+[37, 38].
In our previous study, we have reported that some Ln-MOFs show sensitive response to nitrobenzene through fluorescence quenching mechanism[39]. In continuation to that study, herein we report the synthesis of a new Eu-MOF (1) by a solvothermal method using 1, 3, 5-tri(4-carboxyphenyl)benzene (H3BTB) as ligand for that polycarboxylic acids have rich coordination modes and strong coordination ability[40-43]. 1 is a two-dimensional (2D) laminar structure, and the three-dimensional (3D) structure was formed by the stacking of the 2D layers through π-π interactions. The luminescence explorations revealed that 1 possesses good selectivity and sensitivity for the sensing of Fe3+. Additionally, color tuning in Eu3+/Tb3+ systems was achieved by varying lanthanide ions ratios in the reaction mixture.
2. EXPERIMENTAL
2.1 Materials and instruments
All the chemicals including H3BTB used in this study were of analytical grade and commercially available.
The luminescence spectra and sensing properties for the powdered solid samples were measured on an Edinburgh FS5 spectrophotometer under identical experimental conditions. Fourier transform infrared (FTIR) spectra were obtained on a Tensor 27 OPUS (Bruker) FT-IR spectrometer in a wavenumber range of 400~4000 cm-1. Thermogravimetric (TG) analysis was carried out on a Rigaku standard TG-DTA analyzer at a heating rate of 10 ℃⋅min-1 from ambient temperature to 800 ℃ using an empty Al2O3 crucible as a reference. The powder X-ray diffraction spectra (PXRD) were recorded on a Rigaku D/Max-2500 diffractometer at 40 kV, 100 mA for a Cu-target tube, and a graphite monochromator. Simulation of the PXRD pattern was carried out by the single-crystal data and diffraction-crystal module of the Mercury (Hg) program version 1.4.2. Elemental analyses (C, H and N) were performed on a Perkin-Elemer240C analyzer.
2.2 Synthesis and crystallization
2.2.1 Synthesis of [Eu(BTB)(phen)(DMF)]žDMF (1)
1 was synthesized using solvothermal method. A mixture of Eu(NO3)3·6H2O, H3BTB, 1, 10-phenanthroline, and 5 mL DMF: H2O (v: v = 1:1) was added to a glass bottle, heated at 95 ℃ for 3 days, and then cooled to room temperature. The heterogeneous solution was separated from the solid phase and the crystals were washed with ethanol and dried at room temperature. Colorless block crystals were obtained. The net yield of various components was: 25%. Anal. Calcd. for C45H37EuN4O8 (%): C, 59.14; H, 4.05; N, 6.13. Found (%): C, 59.06; H, 4.15; N, 6.10. FT-IR (KBr pellets, cm-1): 3444 w, 1650 m, 1582 s, 1520 s, 1409 s, 1182 w, 1104 w, 1014 w, 963 w, 859 w, 787 s, 730 w.
2.2.2 Synthesis of [EuxTb1-x(BTB)(phen) (DMF)]žDMF (2~5)
EuxTb1-x-MOF were synthesized according to a similar procedure to that of 1 except doping a certain amount of Tb(NO3)3⋅6H2O instead of Eu(NO3)3⋅6H2O. The percentage of Tb(NO3)3⋅6H2O was 90.91% (2), 98.04% (3), 99.01% (4) and 100% (5), respectively. Complex 2: yield: 23%. Anal. Calcd. for C45H37Eu0.0909Tb0.9091N4O8: C, 58.74%; H, 4.05%; N, 6.09%. Found: C, 58.82%; H, 4.12%; N, 6.15%. FT-IR (KBr pellets, cm-1): 3440 m, 3444 w, 1650 m, 1606 w, 1581 m, 1531 s, 1411 s, 1182 w, 1105 w, 1014 w, 860 m, 786 s, 730 m, 667 w, 470 m. Complex 3: Yield: 24%. Anal. Calcd. for C45H37Eu0.0196Tb0.9804N4O8: C, 58.71%; H, 4.05%; N, 6.09%. Found: C, 58.66%; H, 4.01%; N, 6.02%. FT-IR (KBr pellets, cm-1): 3436 m, 2925 w, 2350 w, 1650 m, 1581 s, 1531 m, 1384 s, 1182 w, 1105 w, 1014 w, 860 m, 788 s, 730 w, 673 m, 470 m. Complex 4: yield: 21%. Anal. Calcd. for C45H37Eu0.0009Tb0.9901N4O8: C, 58.79%; H, 4.06%; N, 6.09%. Found: C, 58.71%; H, 4.13%; N, 6.15%. FT-IR (KBr pellets, cm-1): 3471 m, 3060 w, 2925 w, 1650 m, 1581 m, 1527 m, 1425 s, 1182 w, 1014 w, 860 m, 813 w, 786 s, 730 m, 671 m, 468 m. Complex 5: yield: 25%. Anal. Calcd. for C45H37TbN4O8: C, 58.70%; H, 4.05%; N, 6.09%. Found: C, 58.66%; H, 4.11%; N, 6.17%. FT-IR (KBr pellets, cm-1): 3430 m, 3060 w, 2925 w, 1650 m, 1581 m, 1529 m, 1421 s, 1182 w, 1105 w, 1014 w, 850 m, 706 s, 730 m, 671 m, 468 m.
2.3 X-ray crystallography
X-ray single-crystal diffraction data for 1 were collected on a Rigaku SCX-mini diffractometer at 293(2) K with Mo-Kα radiation (λ = 0.71073 Å) by an ω scan mode. The crystal data were solved by direct methods and refined by full-matrix least-squares technique on F2 using the SHELXS and SHELXL. More details on the crystallographic studies as well as atomic displacement parameters are given in the CIF file. All carbon-bonded hydrogen atoms were added theoretically, riding on the concerning atoms and refined with fixed thermal factors. Crystal data for 1: triclinic system, P
$ \overline 1 $ space group with a = 9.0694(6), b = 14.7630(9), c = 15.9047(7) Å, α = 69.049(5)°, β = 81.891(5)°, γ = 83.602(5)°, V = 1964.5(2) Å3, Z = 2, C45H37EuN4O8, Mr = 913.75, Dc = 1.545 g/cm3, F(000) = 924, GOOF = 0.93. Selected bond lengths and bond angles of 1 are given in Table 1.Table 1
Bond Dist. Bond Dist. Bond Dist. Eu(1)–O(4)i 2.410(7) Eu(1)–O(3)i 2.593(8) Eu(1)–O(5)ii 2.501(8) Eu(1)–O(6)ii 2.428(7) Eu(1)–O(1) 2.462(7) Eu(1)–N(1) 2.591(9) Eu(1)–O(7) 2.467(8) Eu(1)–O(2) 2.494(7) Eu(1)–N(2) 2.583(9) Angle (°) Angle (°) Angle (°) O(4)i–Eu(1)–O(6)ii 123.2(2) O(6)ii–Eu(1)–O(1) 136.2(3) O(1)–Eu(1)–N(1) 86.7(3) O(6)ii–Eu(1)–O(7) 71.2(3) O(4)i–Eu(1)–O(2) 121.3(3) O(2)–Eu(1)–N(1) 83.4(3) O(1)–Eu(1)–O(2) 52.2(3) O(4)i–Eu(1)–O(5)ii 125.2(3) N(2)–Eu(1)–N(1) 63.8(3) O(1)–Eu(1)–O(5)ii 83.2(3) O(6)ii–Eu(1)–O(3)i 73.0(3) O(7)–Eu(1)–O(3)i 135.9(3) O(4)i–Eu(1)–O(1) 75.0(2) O(2)–Eu(1)–O(5)ii 76.5(3) O(5)ii–Eu(1)–O(3)i 85.4(3) O(4)i–Eu(1)–O(7) 140.7(3) O(6)ii–Eu(1)–N(2) 76.8(2) N(1)–Eu(1)–O(3)i 114.0(2) O(1)–Eu(1)–O(7) 122.3(3) O(7)–Eu(1)–N(2) 69.8(3) O(2)–Eu(1)–N(2) 133.0(3) O(6)ii–Eu(1)–O(2) 113.1(3) O(5)ii–Eu(1)–N(2) 130.1(2) O(4)i–Eu(1)–N(1) 68.3(3) O(7)–Eu(1)–O(2) 70.9(3) O(6)ii–Eu(1)–N(1) 136.0(3) O(2)–Eu(1)–O(3)i 149.1(3) O(6)ii–Eu(1)–O(5)ii 53.3(2) O(7)–Eu(1)–N(1) 77.3(3) N(2)–Eu(1)–O(3)i 77.7(3) O(7)–Eu(1)–O(5)ii 93.3(3) O(5)ii–Eu(1)–N(1) 159.7(3) O(1)–Eu(1)–N(2) 145.9(3) O(4)i–Eu(1)–N(2) 78.2(3) O(4)i–Eu(1)–O(3)i 52.1(2) O(1)–Eu(1)–O(3)i 101.4(3) Symmetry codes: (i) x, y – 1, z + 1; (ii) x + 1, y – 1, z 3. RESULTS AND DISCUSSION
3.1 Description of the crystal structure
Single-crystal X-ray diffraction analysis reveals that 1 crystallizes in the triclinic system with space group P
$ \overline 1 $ . In the asymmetric unit, there are one Eu3+ ion, one BTB3- ligand, one 1, 10-phenanthroline (phen) and one coordinated DMF as well as a free DMF. The central Eu3+ ion exhibits a nine-coordinate environment forming a twisted triangular prism with six oxygen atoms from three BTB3-, two nitrogen atoms from a phen and an oxygen atom from a coordinated DMF (Fig. 1a). Each BTB3- connects three Eu3+ ions through chelating coordination of carboxylic acids (Fig. 1b). As shown in Fig. 1c, Eu3+ cations are connected as a node through three BTB3- to form a 2D plane structure. Each 2D stratified structure is stacked into a 3D supramolecular structure through π-π interactions with the shortest centroid-to-centroid distance of 3.646 Å (Fig. 1d). Topologically, Eu3+ and BTB3- can be simplified as 3-connected nodes, and the 2D plane as a hcb net (Fig. 1e).Figure 1
3.2 PXRD and thermogravimetric analysis
The purity of the bulky crystalline samples of 1 was confirmed by PXRD at room temperature. As shown in Fig. 2, the PXRD pattern of the as-synthesized 1 corresponds well with the simulated one based on the single-crystal diffraction data, which confirms the good purity of 1.
Figure 2
The thermal stability of the complex was evaluated by TG experiment and the results shown in Fig. 3 suggest that the first weight loss of about 16.5% (calculated 16.0%) occurred from room temperature to 500 ℃, corresponding to the removal of DMF molecules. Subsequently, there is a quick mass loss at 500~700 ℃, which may correspond to the decomposition of the framework.
Figure 3
3.3 Fluorescence properties
3.3.1 Solid-state photoluminescent spectra
The luminescent spectra of 1 were determined in the solid state at room temperature and the results are shown in Fig. 4. When excited at 300 nm, the emission peaks at 579, 588, 594, 615, 618, 650, 683 and 692 nm correspond to the transition from 5D0 → 7FJ (J = 0~4) of Eu3+ ions. The most intense emissions in the luminescent spectra around 615 and 618 nm are ascribed to the electric dipolar 5D0 → 7F2 transition, which is responsible for the red emission observed for these MOFs[44]. The splitting emission band for the 5D0→7F1, 5D0→7F2 and 5D0→7F4 transition indicates only one type of coordination environment for the Eu3+ cation in 1[44]. The results are in good agreement with the single-crystal X-ray analyses.
Figure 4
3.3.2 Fluorescence sensing properties of 1
The fluorescence properties of 1 in different metal ion solutions were also investigated. The corresponding MOF/Mm+ was prepared by introducing 1 (3 mg) powder into 3 mL ethanol solution of MNOx (Mm+ = K+, Cd2+, Mg2+, Mn2+, Ni2+, Ca2+, Zn2+, Cu2+, Fe3+) with each concentration to be 1 mmol/L, which was uniformly dispersed under ultrasonication for 5 min. A series of luminescent responses were recorded and compiled in Fig. 5a, which suggests that at 615 nm, 1 exhibited luminescence intensities for different metal ions with great degree of variation in their bursting effect. Interestingly, Fig. 5b shows that 1 exhibits a drastic quenching effect in the Fe3+ ion solution, which can be of great help in practical application for the sensing of Fe3+, which was further evaluated in the subsequent experiments. Due to the coexistence of multiple metal ions in real solution system, competing experiments were conducted with respectively adding various metal ions to the suspension of 1, followed by the treatment of equivalent amount of Fe3+. As can be seen from Fig. 5c, the presence of other metal ions has almost no significant effect on the selectivity of 1 to Fe3+, further indicating the high fluorescence selectivity of 1 to Fe3+.
Figure 5
Figure 5. (a) Luminescence spectra of 1 dispersed in ethanol solutions of different metal ions; (b) Maximum emission intensity of 1 in multiple metal cation-ethanol solution; (c) Luminescence intensity (615 nm) of 1 dispersed in EtOH with the addition of different ions (Mg2+, Cd2+, Zn2+, Mn2+, K+, Ni2+, Ca2+, Cu2+) (1 mmol/L) and Fe3+ incorporated systems (1 mmol/L)To better understand the sensing sensitivities of 1 for Fe3+ ions, a series of titrating experiments were carried out in ethanol solutions with variable concentrations of Fe3+ ions. 1 (3 mg) was sonicated in EtOH (3 mL) followed by the addition of different volumes of Fe3+ (1 mmol/L) and the emission spectra were measured as displayed in Fig. 6. It is noted that the fluorescence intensity of 1 is completely quenched at Fe3+ ion concentration of 0.1 mM (300 µL). The relationship between the quenching effect and Fe3+ concentration was further investigated through the Stern-Volmer equation i.e. I0/I = 1 + KSV [M], where I0 and I represent the initial luminescence intensity and luminescence intensity after adding analyte, respectively, [M] is the concentration of analyte, and KSV stands for the Stern-Volmer constant[45]. The Stern-Volmer plot of 1 in Fig. 7 shows that at higher Fe3+ ion concentration, there is a nonlinear upward curvature, indicating that the mechanism of luminescence quenching may be a combination of dynamic quenching and static quenching[32]. The KSV value was found to be 3.91 × 104 M-1 for 1 at low concentrations of Fe3+ ions. The value is comparable with those reported for MOF-based sensor materials in the literatures (Table 2)[46-49]. The limits of detection (LOD) can be determined by LOD = 3σ/k (k is the slope of the linear curve and σ is the standard deviation of the blank sample)[50]. With the slope of the fitting line and the measurement error of the intensity with blank samples, the detection limit for Fe3+ was calculated to be 1.7 μM. On the other hand, the quenching efficiency of 1 can be maintained above 80% through five cycles of experiments (Fig. 8).
Figure 6
Figure 7
Table 2
Complex KSV (M-1) Ref. {Ni(1, 4-bib)1.5(TPA-Cl2)·H2O}n 7×104 [46] JLU-MOF71 5.77×104 [47] [Zn4(bptc)2(NMP)3(DMF)(H2O)2]n 4.12×103 [48] [Cd8(bptc)4(NMP)6(DMF)4(H2O)2]n 6.57×103 [48] {[Zn2(bptc)(DMA)(H2O)2]·(DMA)2·H2O}n 3.76×103 [48] {[CoL2Cl2]ž4CH3OH}∞ 1.07×104 [49] {[NiL2Cl2]ž4CH3OH}∞ 1.67×104 [49] {[CoL2Br2]ž4CH3OH}∞ 3.33×104 [49] Eu-MOF 3.91 ×104 This work Figure 8
3.4 Color tuning
Because of the similar coordination environment and coordination number of the Ln3+ ions, different Ln ions can be combined in the same MOF. Using an analogous synthetic protocol to that used for the preparation of 1, various ratios of Eu3+ and Tb3+ were introduced to the solutions of H3BTB in DMF: water and various complexes of EuxTb1-x were synthesized (complexes 2~5). After isolation of the crystalline products, PXRD were performed to analyze the structural analogy of these complexes to homometallic 1 (Fig. 9). The solid samples were then studied by fluorescence spectroscopy and the effect of altering the lanthanide ion ratios in EuxTb1-x was manifested in the variation of the emission spectra (Fig. 10). It was noted that as the molar ratio of Eu3+ increased from 0.0009 to 0.0909, the relative emission intensity of the strongest emission of Tb3+ at 544 nm gradually weakened, while that of the Eu3+ ion at 615 nm gradually enhanced. The general shift in color can be safely assumed giving the trend of the spectra, and chromaticity coordinates were calculated and plotted in the CIE coordinate diagram to more clearly present the colors of emission (Fig. 11).
Figure 9
Figure 10
Figure 11
4. CONCLUSION
In summary, a novel 2D Eu-MOF (1) was successfully synthesized under solvothermal conditions using H3BTB as the ligand. X-ray single-crystal analysis demonstrated that 1 is a 3D supramolecular network formed by the stacking of 2D layers through π-π interactions. The luminescence explorations revealed that 1 possesses high selectivity and sensitivity for the sensing of Fe3+ by quenching mechanism with KSV = 3.91 × 104 M-1. Furthermore, a series of co-doped Ln-MOFs with tunable luminescence were also fabricated and tested, which showed that their luminescent color can be effectively tuned from red to green by controlling the ratios of Eu3+: Tb3+. This study provides important guidelines for the fabrication of Eu-MOF for the highly efficient sensing of Fe3+ in practical applications.
-
-
[1]
Cheng, X. F.; Hu, J. S.; Li, J. X.; Zhang, M. D. Tunable emission and selective luminescence sensing for nitro-pollutants and metal ions based on bifunctional lanthanide metal-organic frameworks. J. Lumin. 2020, 221, 117100−10. doi: 10.1016/j.jlumin.2020.117100
-
[2]
Yao, S. L.; Xiong, Y. C.; Tian, X. M.; Liu, S. J.; Xu, H.; Zheng, T. F.; Chen, J. L.; Wen, H. R. A multifunctional benzothiadiazole-based fluorescence sensor for Al3+, Cr3+ and Fe3+. CrystEngComm 2021, 23, 1898−1905. doi: 10.1039/D1CE00060H
-
[3]
Yao, Q. X.; Tian, M. M.; Wang, Y.; Meng, Y. J.; Wang, J.; Yao, Q. Y.; Zhou, X.; Yang, H.; Wang, H. W.; Li, Y. W.; Zhang, J. A robust, water-stable, and multifunctional praseodymium-organic framework showing permanent porosity, CO2 adsorption properties, and selective sensing of Fe3+ ion. Chin. J. Struct. Chem. 2020, 39, 1862−1870.
-
[4]
Chandra Rao, P.; Mandal, S. Europium-based metal-organic framework as a dual luminescence sensor for the selective detection of the phosphate anion and Fe3+ ion in aqueous media. Inorg. Chem. 2018, 57, 11855−11858. doi: 10.1021/acs.inorgchem.8b02017
-
[5]
Zhang, Z.; Fang, Q. H.; Zhuang, Z. Y.; Han, Y.; Li, L. Y.; Yu, Y. Europium activated aluminum organic frameworks for highly selective and sensitive setection of Fe3+ and Cr(Ⅵ) in aqueous solution. Chin. J. Struct. Chem. 2020, 39, 1958−1964.
-
[6]
Guo, X. H.; Li, Y. S.; Peng, Q. Y.; Duan, Z. M.; Li, M. X.; Shao, M.; He, X. Dual functional three-dimensional LnMOFs for luminescence sensing of nitrobenzene and Fe3+ ions. Polyhedron 2017, 133, 238−244. doi: 10.1016/j.poly.2017.04.033
-
[7]
Wu, N.; Guo, H.; Wang, X. Q.; Sun, L.; Zhang, T. T.; Peng, L. P.; Yang, W. A water-stable lanthanide-MOF as a highly sensitive and selective luminescence sensor for detection of Fe3+ and benzaldehyde. Colloids Surf. A: Physicochem. Eng. Asp. 2021, 616, 126093−7. doi: 10.1016/j.colsurfa.2020.126093
-
[8]
Guan, B. B.; Li, Q.; Xu, Y. T.; Chen, L. H.; Wu, Z. S.; Fan, Z. L.; Zhu, W. Highly selective and sensitive detection towards cationic Cu2+ and Fe3+ contaminants via an In-MOF based dual-responsive fluorescence probe. Inorg. Chem. Commun. 2020, 122, 108273−7. doi: 10.1016/j.inoche.2020.108273
-
[9]
Xia, Z. Q.; Ren, C. T.; Xu, W. F.; Li, F.; Qiao, C. F.; Wei, Q.; Zhou, C. S.; Chen, S. P.; Gao, S. L. Ultrasensitive Fe3+ luminescence sensing and supercapacitor performances of a triphenylamine-based TbIII-MOF. J. Solid State Chem. 2020, 282, 121083−7. doi: 10.1016/j.jssc.2019.121083
-
[10]
Yin, X. J.; Li, S. X.; Liao, B. L. Water-stable Ln-exclusive metal-organic framework for highly selective sensing of Fe3+ ions. Dyes Pigm. 2020, 174, 108035−7. doi: 10.1016/j.dyepig.2019.108035
-
[11]
Yu, M. H.; Liu, X. T.; Space, B.; Chang, Z.; Bu, X. H. Metal-organic materials with triazine-based ligands: from structures to properties and applications. Coord. Chem. Rev. 2021, 427, 213518−45. doi: 10.1016/j.ccr.2020.213518
-
[12]
Wang, C. C.; Wang, X.; Liu, W. The synthesis strategies and photocatalytic performances of TiO2/MOFs composites: a state-of-the-art review. Chem. Eng. J. 2020, 391, 1385−8947.
-
[13]
Kong, L. J.; Liu, M.; Huang, H.; Xu, Y. H.; Bu, X. H. Metal/covalent-organic framework based cathodes for metal-ion batteries. Adv. Energy Mater. 2021, 2100172−26.
-
[14]
Xiao, Q. Q.; Dong, G. Y.; Li, Y. H.; Cui, G. H. Cobalt(II)-based 3D coordination polymer with unusual 4, 4, 4-connected topology as a dual-responsive fluorescent chemosensor for acetylacetone and Cr2O72-. Inorg. Chem. 2019, 58, 15696−15699. doi: 10.1021/acs.inorgchem.9b02534
-
[15]
Zhu, H.; Li, Y. H.; Xiao, Q. Q.; Cui, G. H. Three luminescent Cd(II) coordination polymers containing aromatic dicarboxylate and flexible bis(benzimidazole) ligands as highly sensitive and selective sensors for detection of Cr2O72- oxoanions in water. Polyhedron 2020, 187, 114648−8. doi: 10.1016/j.poly.2020.114648
-
[16]
Liu, A. J.; Xu, F.; Han, S. D.; Pan, J.; Wang, G. M. Mixed-ligand strategy for the construction of photochromic metal-organic frameworks driven by electron-transfer between nonphotoactive units. Cryst. Growth Des. 2020, 20, 7350−7355. doi: 10.1021/acs.cgd.0c01018
-
[17]
Chen, D. D.; Yi, X. H.; Wang, C. C. Preparation of metal-organic frameworks and their composites using mechanochemical methods. Chin. J. Inorg. Chem. 2020, 36, 1805−1821.
-
[18]
Ju, P.; Liu, X. C.; Zhang, E. S. A novel 3D Zn-based luminescence metal-organic framework: synthesis, structure and fluorescence enhanced sensing of ammonia vapor in air. Chin. J. Struct. Chem. 2020, 39, 1458−1464.
-
[19]
Rocha, J.; Carlos, L. D.; Paz, F. A.; Ananias, D. Luminescent multifunctional lanthanides-based metal-organic frameworks. Chem. Soc. Rev. 2011, 40, 926−940. doi: 10.1039/C0CS00130A
-
[20]
Zhai, L. J.; Jiao, C. X.; Liang, T. F.; Zhang, J.; Niu, X. Y.; Hu, T. P.; Niu, Y. L. Two new coordination polymers based on H4BIPA-TC: syntheses and fluorescence sensing for nitroaromatic compounds and Fe3+ ion. Chin. J. Struct. Chem. 2020, 39, 772−782.
-
[21]
Lv, X. L.; Feng, L.; Wang, K. Y.; Xie, L. H.; He, T.; Wu, W.; Li, J. R.; Zhou, H. C. A series of mesoporous rare-earth metal-organic frameworks constructed from organic secondary building units. Angew. Chem. Int. Ed. 2021, 60, 2053−2057. doi: 10.1002/anie.202011653
-
[22]
Lv, X. L.; Feng, L.; Xie, L. H.; He, T.; Wu, W.; Wang, K. Y.; Si, G. R.; Wang, B.; Li, J. R.; Zhou, H. C. Linker desymmetrization: access to a series of rare-earth tetracarboxylate frameworks with eight-connected hexanuclear nodes. J. Am. Chem. Soc. 2021, 143, 2784−2791. doi: 10.1021/jacs.0c11546
-
[23]
Kumar, M.; Li, L. Q.; Zareba, J. K.; Tashi, L.; Sahoo, S. C.; Nyk, M.; Liu, S. J.; Sheikh, H. N. Lanthanide contraction in cction: structural variations in 13 lanthanide(III) thiophene-2, 5-dicarboxylate coordination polymers (Ln = La-Lu, except Pm and Tm) featuring magnetocaloric effect, slow magnetic relaxation, and luminescence-lifetime-based thermometry. Cryst. Growth Des. 2020, 20, 6430−6452. doi: 10.1021/acs.cgd.0c00611
-
[24]
Li, Y. P.; Yang, H. R.; Zhao, Q.; Song, W. C.; Han, J.; Bu, X. H. Ratiometric and selective fluorescent sensor for Zn2+ as an "off-on-off" switch and logic gate. Inorg. Chem. 2012, 51, 9642−9648. doi: 10.1021/ic300738e
-
[25]
D'Vries, R. F.; Gomez, G. E.; Mondragon, L. P.; Onna, D.; Barja, B. C.; Soler-Illia, G. J. A. A.; Ellena, J. 1D lanthanide coordination polymers based on lanthanides and 4΄-hydroxi-4-biphenylcarboxylic acid: synthesis, structures and luminescence properties. J. Solid State Chem. 2019, 274, 322−328. doi: 10.1016/j.jssc.2019.02.043
-
[26]
Yang, Y.; Wang, Y.; Feng, Y.; Song, X.; Cao, C.; Zhang, G.; Liu, W. Three isostructural Eu3+/Tb3+ co-doped MOFs for wide-range ratiometric temperature sensing. Talanta 2020, 208, 120354−6. doi: 10.1016/j.talanta.2019.120354
-
[27]
Zhou, X. J.; Chen, L. N.; Feng, Z. S.; Jiang, S.; Lin, J. Z.; Pang, Y.; Li, L.; Xiang, G. T. Color tunable emission and low-temperature luminescent sensing of europium and terbium carboxylic acid complexes. Inorg. Chim. Acta 2018, 469, 576−582. doi: 10.1016/j.ica.2017.10.014
-
[28]
Zhu, Y. Y.; Xia, T. F.; Zhang, Q.; Cui, Y. J.; Yang, Y.; Qian, G. D. A Eu/Tb mixed lanthanide coordination polymer with rare 2D thick layers: synthesis, characterization and ratiometric temperature sensing. J. Solid State Chem. 2018, 259, 98−103. doi: 10.1016/j.jssc.2018.01.009
-
[29]
Pang, X. L.; Yu, T.; Shen, F. J.; Yu, X. D.; Li, Y. J. Fluorescence sensing of fluoride ions and N, N-dimethylformamide by novel luminescent lanthanide(III) xerogels. J. Lumin. 2018, 204, 169−175. doi: 10.1016/j.jlumin.2018.08.004
-
[30]
Zhai, B.; Xu, H.; Li, Z. Y.; Cao, C. S.; Zhao, B. A water-stable metal-organic framework: serving as a chemical sensor of PO43– and a catalyst for CO2 conversion. Sci. China. Chem. 2017, 60, 1328−1333.
-
[31]
Chen, D. M.; Zheng, Y. P.; Fang, S. M. A polyhedron-based porous Tb(III)-organic framework with dual emissions for highly selective detection of Al3+ ion. Inorg. Chem. Commun. 2020, 117, 107967−21. doi: 10.1016/j.inoche.2020.107967
-
[32]
Guo, H.; Wu, N.; Xue, R.; Liu, H.; Li, L.; Wang, M. Y.; Yao, W. Q.; Li, Q.; Yang, W. Multifunctional Ln-MOF luminescent probe displaying superior capabilities for highly selective sensing of Fe3+ and Al3+ ions and nitrotoluene. Colloids Surf. A: Physicochem. Eng. Asp. 2020, 585, 124094−8. doi: 10.1016/j.colsurfa.2019.124094
-
[33]
Liang, G. M.; Wang, S.; Xu, M. Y.; Chen, H. L.; Liang, G. Y.; Gui, L. C.; Wang, X. J. 2D lanthanide coordination polymers constructed from a semi-rigid tricarboxylic acid ligand: crystal structure, luminescence sensing and color tuning. CrystEngComm 2020, 22, 6161−6169. doi: 10.1039/D0CE00968G
-
[34]
Chen, D. M.; Sun, C. X.; Peng, Y.; Zhang, N. N.; Si, H. H.; Liu, C. S.; Du, M. Ratiometric fluorescence sensing and colorimetric decoding methanol by a bimetallic lanthanide-organic framework. Sensor Actuat. B-Chem. 2018, 265, 104−109. doi: 10.1016/j.snb.2018.03.028
-
[35]
Zhan, C.; Ou, S.; Zou, C.; Zhao, M.; Wu, C. D. A luminescent mixed-lanthanide-organic framework sensor for decoding different volatile organic molecules. Anal. Chem. 2014, 86, 6648−6653. doi: 10.1021/ac5013442
-
[36]
Zhou, J.; Li, H.; Zhang, H.; Li, H.; Shi, W.; Cheng, P. A bimetallic lanthanide metal-organic material as a self-calibrating color-gradient luminescent sensor. Adv. Mater. 2015, 27, 7072−7077. doi: 10.1002/adma.201502760
-
[37]
Zhang, F. X.; Li, J. Y.; Zhao, Z. R.; Wang, F. Q.; Pu, Y. Y.; Cheng, H. L. Mixed-LnMOFs with tunable color and white light emission together with multi-functional fluorescence detection. J. Solid State Chem. 2019, 280, 120972−5. doi: 10.1016/j.jssc.2019.120972
-
[38]
Yang, Q. Y.; Pan, M.; Wei, S. C.; Li, K.; Du, B. B.; Su, C. Y. Linear dependence of photoluminescence in mixed Ln-MOFs for color tunability and barcode application. Inorg. Chem. 2015, 54, 5707−5716. doi: 10.1021/acs.inorgchem.5b00271
-
[39]
Zhao, S.; Hao, X. M.; Liu, J. L.; Wu, L. W.; Wang, H.; Wu, Y. B.; Yang, D.; Guo, W. L. Construction of Eu(III)- and Tb(III)-MOFs with photoluminescence for sensing small molecules based on furan-2, 5-dicarboxylic acid. J. Solid State Chem. 2017, 255, 76−81. doi: 10.1016/j.jssc.2017.07.039
-
[40]
Dzesse T, C. N.; Nfor, E. N.; Bourne, S. A. Tripodal carboxylate MOFs with Co(II): transmetallation and gas sorption studies. Polyhedron 2020, 189, 114724−8. doi: 10.1016/j.poly.2020.114724
-
[41]
Wu, Y. P.; Tian, J. W.; Liu, S.; Li, B.; Zhao, J.; Ma, L. F.; Li, D. S.; Lan, Y. Q.; Bu, X. Bi-microporous metal-organic frameworks with cubane [M4(OH)4 ] (M = Ni, Co) clusters and pore-space partition for electrocatalytic methanol oxidation reaction. Angew. Chem. Int. Ed. 2019, 58, 12185−12189. doi: 10.1002/anie.201907136
-
[42]
Guo, X. Y.; Zhao, F.; Liu, H. T.; Wang, Y. Q.; Liu, Z. L.; Gao, E. Q. Five new 2D and 3D coordination polymers based on two new multifunctional pyridyl-tricarboxylate ligands: hydrothermal syntheses, structural diversity, luminescent and magnetic properties. RSC Adv. 2017, 7, 19039−19049. doi: 10.1039/C7RA02063E
-
[43]
He, K. H.; Li, Y. W.; Chen, Y. Q.; Song, W. C.; Bu, X. H. Employing zinc clusters as SBUs to construct (3, 8) and (3, 14)-connected coordination networks: structures, topologies, and luminescence. Cryst. Growth Des. 2012, 12, 2730−2735 doi: 10.1021/cg300218z
-
[44]
Zhang, J.; Zheng, B.; Zhao, T. T.; Li, G. H.; Huo, Q. S.; Liu, Y. L. Topological diversities and luminescent properties of lanthanide metal-organic frameworks based on a tetracarboxylate ligand. Cryst. Growth Des. 2014, 14, 2394−2400. doi: 10.1021/cg500114k
-
[45]
Hou, L. L.; Song, Y. H.; Lang, F. X.; Wang, Z. R.; Wang, L. Fluorometric determination of Fe3+ and polychlorinated benzenes based on Tb3+-pyromellitic acid coordination polymer. J. Ind. Eng. Chem. 2020, 90, 145−151. doi: 10.1016/j.jiec.2020.07.006
-
[46]
Cui, J. W.; Hou, S. X.; Li, Y. H.; Cui, G. H. A multifunctional Ni(Ⅱ) coordination polymer: synthesis, crystal structure and applications as a luminescent sensor, electrochemical probe, and photocatalyst. Dalton Trans. 2017, 46, 16911−16924. doi: 10.1039/C7DT03874G
-
[47]
Yu, C.; Sun, X.; Zou, L.; Li, G.; Zhang, L.; Liu, Y. A pillar-layered Zn-LMOF with uncoordinated carboxylic acid sites: high performance for luminescence sensing Fe3+ and TNP. Inorg. Chem. 2019, 58, 4026−4032. doi: 10.1021/acs.inorgchem.9b00204
-
[48]
Huang, W. H.; Ren, J.; Yang, Y. H.; Li, X. M.; Wang, Q.; Jiang, N.; Yu, J. Q.; Wang, F.; Zhang, J. Water-stable metal-organic frameworks with selective sensing on Fe3+ and nitroaromatic explosives, and stimuli-responsive luminescence on lanthanide encapsulation. Inorg. Chem. 2019, 58, 1481−1491. doi: 10.1021/acs.inorgchem.8b02994
-
[49]
Nandi, S.; Reinsch, H.; Banesh, S.; Stock, N.; Trivedi, V.; Biswas, S. Rapid and highly sensitive detection of extracellular and intracellular H2S by an azide-functionalized Al(Ⅲ)-based metal-organic framework. Dalton Trans. 2017, 46, 12856−12864. doi: 10.1039/C7DT02293J
-
[50]
He, T. S.; Lan, Y. L.; Li, Z. Y.; Zhu, L. N.; Li, X. Z. Chiral coordination polymers from a new 2-deoxy-d-ribose derivative linker: syntheses, structures, and Fe3+ fluorescent probe functions. Cryst. Growth Des. 2021, 21, 2233−2242. doi: 10.1021/acs.cgd.0c01661
-
[1]
-
Figure 5 (a) Luminescence spectra of 1 dispersed in ethanol solutions of different metal ions; (b) Maximum emission intensity of 1 in multiple metal cation-ethanol solution; (c) Luminescence intensity (615 nm) of 1 dispersed in EtOH with the addition of different ions (Mg2+, Cd2+, Zn2+, Mn2+, K+, Ni2+, Ca2+, Cu2+) (1 mmol/L) and Fe3+ incorporated systems (1 mmol/L)
Table 1. Selected Bond Lengths (Å) and Bond Angles (°) for 1
Bond Dist. Bond Dist. Bond Dist. Eu(1)–O(4)i 2.410(7) Eu(1)–O(3)i 2.593(8) Eu(1)–O(5)ii 2.501(8) Eu(1)–O(6)ii 2.428(7) Eu(1)–O(1) 2.462(7) Eu(1)–N(1) 2.591(9) Eu(1)–O(7) 2.467(8) Eu(1)–O(2) 2.494(7) Eu(1)–N(2) 2.583(9) Angle (°) Angle (°) Angle (°) O(4)i–Eu(1)–O(6)ii 123.2(2) O(6)ii–Eu(1)–O(1) 136.2(3) O(1)–Eu(1)–N(1) 86.7(3) O(6)ii–Eu(1)–O(7) 71.2(3) O(4)i–Eu(1)–O(2) 121.3(3) O(2)–Eu(1)–N(1) 83.4(3) O(1)–Eu(1)–O(2) 52.2(3) O(4)i–Eu(1)–O(5)ii 125.2(3) N(2)–Eu(1)–N(1) 63.8(3) O(1)–Eu(1)–O(5)ii 83.2(3) O(6)ii–Eu(1)–O(3)i 73.0(3) O(7)–Eu(1)–O(3)i 135.9(3) O(4)i–Eu(1)–O(1) 75.0(2) O(2)–Eu(1)–O(5)ii 76.5(3) O(5)ii–Eu(1)–O(3)i 85.4(3) O(4)i–Eu(1)–O(7) 140.7(3) O(6)ii–Eu(1)–N(2) 76.8(2) N(1)–Eu(1)–O(3)i 114.0(2) O(1)–Eu(1)–O(7) 122.3(3) O(7)–Eu(1)–N(2) 69.8(3) O(2)–Eu(1)–N(2) 133.0(3) O(6)ii–Eu(1)–O(2) 113.1(3) O(5)ii–Eu(1)–N(2) 130.1(2) O(4)i–Eu(1)–N(1) 68.3(3) O(7)–Eu(1)–O(2) 70.9(3) O(6)ii–Eu(1)–N(1) 136.0(3) O(2)–Eu(1)–O(3)i 149.1(3) O(6)ii–Eu(1)–O(5)ii 53.3(2) O(7)–Eu(1)–N(1) 77.3(3) N(2)–Eu(1)–O(3)i 77.7(3) O(7)–Eu(1)–O(5)ii 93.3(3) O(5)ii–Eu(1)–N(1) 159.7(3) O(1)–Eu(1)–N(2) 145.9(3) O(4)i–Eu(1)–N(2) 78.2(3) O(4)i–Eu(1)–O(3)i 52.1(2) O(1)–Eu(1)–O(3)i 101.4(3) Symmetry codes: (i) x, y – 1, z + 1; (ii) x + 1, y – 1, z Table 2. KSV Values for Fe3+ in Comparison with Literatures
Complex KSV (M-1) Ref. {Ni(1, 4-bib)1.5(TPA-Cl2)·H2O}n 7×104 [46] JLU-MOF71 5.77×104 [47] [Zn4(bptc)2(NMP)3(DMF)(H2O)2]n 4.12×103 [48] [Cd8(bptc)4(NMP)6(DMF)4(H2O)2]n 6.57×103 [48] {[Zn2(bptc)(DMA)(H2O)2]·(DMA)2·H2O}n 3.76×103 [48] {[CoL2Cl2]ž4CH3OH}∞ 1.07×104 [49] {[NiL2Cl2]ž4CH3OH}∞ 1.67×104 [49] {[CoL2Br2]ž4CH3OH}∞ 3.33×104 [49] Eu-MOF 3.91 ×104 This work -

计量
- PDF下载量: 2
- 文章访问数: 292
- HTML全文浏览量: 5