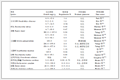

Influence of Doped Ions on Persistent Luminescence Materials: a Review
English
Influence of Doped Ions on Persistent Luminescence Materials: a Review
-
Key words:
- persistent luminescence
- / doped ions
- / structure and optical performance
-
1. INTRODUCTION
Persistent luminescence materials (PLMs) can absorb excitation energy and continue to emit light after the excitation is stopped[1-7]. In ancient China, natural minerals with luminous properties were made into luminous cups and night pearls. In 1866, Sidot et al. first prepared the sulfide PLMs ZnS: Cu2+, making PLMs enter the vision of researchers[8]. In 1968, Pailil et al. observed the persistent luminescence of SrAl2O4: Eu2+, pushing the researches of PLMs into a new stage[9, 10]. Subsequently, Matsuzawa et al. found that the phosphorescence intensity and phosphorescence time of SrAl2O4: Eu2+, Dy3+ phosphors were more than ten times those of early PLMs[11]. Since then, many PLMs have been developed and widely used in transportation, military facilities, biosensing and other fields[12-15].
The persistent luminescence inherence makes PLMs proper candidates in material applications. Given this, many researchers are devoted to finding ways to improve the phosphorescence intensity and phosphorescence time of PLMs. The introduction of doped ions provides a possibility to improve the optical performance of PLMs[16-23]. For example, Yamamoto et al. discovered that the phosphorescence intensity and phosphorescence time of the materials were improved after introducing Dy3+ ion into SrAl2O4: Eu2+[11]. Liu et al. enhanced the phosphorescence intensity and prolonged the phosphorescence time exceeding 13 hours by adjusting the contents of Nd3+ ion in Zn2Ga3–x–yGe0.75O8: Crx, Ndy[24]. Studying the mechanisms of ion doping to enhance the optical performance of PLMs can help design PLMs with stronger phosphorescence intensity and longer phosphorescence time, thereby expanding the applcations of PLMs.
Recently, reviews focus on synthesis methods, persistent luminescence mechanisms and applications of PLMs have been published. Li et al. systematically summarized the synthesis techniques, persistent luminescence mechanisms, characterizations and applications of PLMs[9]. Wang et al. summarized the persistent luminescence mechanisms, synthesis methods and biomedical applications of PLMs[25]. Singh et al. outlined the surface modifications, bioimaging applications of PLMs, and future research directions[26]. Doped ions play a vital role in improving the optical performance of PLMs. However, the influence of doped ions on the structure and optical performance of PLMs is seldom summarized. In this review, we mainly focus on how the optical performance of PLMs is affected by the type of doped ions, the number of types of doped ions, and the content of doped ions. Furthermore, the opportunities and challenges of PLMs in ion doping are also presented.
2. INFLUENCE OF DOPED IONS ON THE STRUCTURE AND OPTICAL PERFORMANCE OF PLMs
With the introduction of doped ions, different energy levels are produced in PLMs, resulting in different structures and optical performance. Herein, we classify and discuss how doped ions affect the structure and optical performance of PLMs based on the number of types of doped ions, the type of doped ions and the content of doped ions. To better demonstrate the influence of doped ions on PLMs, a summary of the main influence of doped ions on some common ion-doped PLMs is shown in Table 1.
Table 1
Host Emitter Co-dopants Emission region Afterglow decay Ref. SrAl2O4 Eu2+ 450~700 nm > 2 h 4 GdAlO3 Mn2+ Ge4+ 600~840 nm > 1200 s 64 Y3Al5–xGaxO12 (x = 0~4) Ce3+ Bi3+ 505 nm 54~68 min 67 ZnGa2O4 B3+ 502, 695 nm 600 s 18 Zn1.25Ga1.5Ge0.25O4 Cr3+ Yb3+, Er3+ 650~850 nm > 480 h 23 Li2ZnGeO4 Mn2+ 550~800 nm > 960 h 62 MgGeO3 Mn2+ Bi3+ 680 nm 100 min 68 Y3Sc2Ga3O12 Ce3+ 500 nm 100 min 69 CaMgSi2O6 Eu2+ Dy3+, Mn2+ 468, 600, 670 nm > 600s 66 Ca9Bi(PO4)7 Ce3+ Tb3+, Mn2+ 300~500 nm 5 h 70 Ca2BO3Cl Eu2+ Dy3+ 580 nm 48 h 71 Gd2O2S Eu3+ Ti4+, Mg2+ 580~720 nm 120 s 20 Lu2O3 Tb3+ Ca2+ 543 nm 20~30 h 72 KY3F10 Tb3+ 542 nm 108 s 73 AlN Mn2+ 570~700 nm 1 h 74 2.1 Influence of doped ions type on the structure and optical performance of PLMs
Due to the difference in the atomic structure and energy levels, various doped ions have different effects on the structure and optical performance of PLMs. Thus, the relationship between the type of doped ions and the structure and optical performance of PLMs is introduced in this section.
2.1.1 Cr3+-doped PLMs
Cr3+ ion is usually introduced into PLMs to obtain red or near-infrared persistent luminescence resulting from its unique electronic transitions. Among them, Cr3+-doped ZnGa2O4 is one of the research hotspots in PLMs. The ZnGa2O4 crystal exhibits a cubic spinel structure, in which the Zn2+ ion occupies the A-sites of the tetrahedron, and the Ga3+ ion the B-sites of the octahedron[27, 28]. Cr3+ ion tends to replace Ga3+ ion in the distorted octahedral coordination for the reason that Cr3+ ion has the same valence electron and ion radius as Ga3+ ion, which makes the Cr3+-doped ZnGa2O4 materials produce strong near-infrared phosphorescence at 696 nm[2, 29, 30]. Hao et al. found that the Cr3+-doped ZnGa2O4 exhibits excellent optical performance, which can maintain phosphorescence for 10 hours after stopping the ultraviolet light irradiation[31]. Moreover, the author observed that the excitation spectrum of Cr3+-doped ZnGa2O4 has three excitation bands of 250~350, 350~487, and 487~650 nm, which are caused by the host excitation band of ZnGa2O4, the charge transfer between ZnGa2O4 and Cr3+ ion, and the electronic transitions of Cr3+ ion, respectively. Based on previous researches, Yan et al. further comprehensively studied the persistent luminescence mechanism of Zn2.94Ga1.96Ge2O10: Cr3+[19]. As shown in Fig. 1, after the Zn2.94Ga1.96Ge2O10 absorbs incident photons, the electrons of Zn2.94Ga1.96Ge2O10 move to the conduction band and are trapped by native defects by means of nonradiative relaxation. When the ultraviolet light excitation is finished, the combination of holes and electrons released by native defects produces short phosphorescence. Since the absorption spectrum of Cr3+ ion fully overlaps with the emission spectrum of Zn2.94Ga1.96Ge2O10, the energy absorbed by Zn2.94Ga1.96Ge2O10 is transferred to Cr3+ ion by means of nonradiative energy[32]. The continuous energy transfer causes the electrons of Cr3+ ion to transform into three different excited states. Then, the electrons are trapped by the shallow electron traps or deep electron traps by means of nonradiative relaxation[33]. At the same time, the electron (t2e) fills the energy-matched traps in the form of 4T1 and 4T2 through the tunneling process. When the continuous energy transfer is stopped, the electron traps release the electrons which recombine with Cr3+ ion, thereby producing a strong or ultra-long phosphorescence[19].
Figure 1
2.1.2 Mn2+-doped PLMs
Mn2+ ion has a typical 3d5 electronic configuration. The energy transitions of Mn2+ ions are quite sensitive to the ligand/crystal field due to the participation of the d shell. Mn2+ ions surrounded by anions can have different geometric structures, such as linear, octahedron, spherical, tetrahedron, or square plane. The environment of Mn2+ ion at different crystal fields leads to different emission energy distributions and produces an emission band of 450~750 nm. For example, tetrahedral coordination of Mn2+ ion produces green emission[34], while octahedral coordination of Mn2+ ion produces orange to red emission[35, 36]. Tan et al. researched the size, crystal structure and optical performance of Mn2+-doped ZnGa2O4 with a typical rod-shaped structure in different environments[21]. The size of Mn2+-doped ZnGa2O4 rapidly decreases as the pH increases from 6 to 7.5. In comparison, when the pH further increases to 9.5, the size of the Mn2+-doped ZnGa2O4 materials slightly increases to 80 nm. The detailed crystal structure of the Mn2+-doped ZnGa2O4 shows that the materials have lattice fringe (110) parallel to the direction of the crystal rod and lattice fringe (113) at an angle of 66° to the direction of the crystal rod[37]. Their interplanar spacing is 0.71 and 0.29 nm, indicating that the Mn2+-doped ZnGa2O4 grows along the c axis[38]. The optical performance of ZnGa2O4: Mn2+ materials was further researched by researchers[39]. The photoluminescence spectrum of Mn2+-doped ZnGa2O4 materials shows that two emission peaks are detected at 450 and 480 nm, which are resulted from the native defects, for example, interstitial Zn and oxygen vacancies (Fig. 2a). When the pH is below 7.0, the intrinsic luminescence of the materials and the emission of Mn2+ ions have a serious overlap. As the pH increases, the intrinsic luminescence intensity gradually decreases, and the emission band intensity of the Mn2+ ion increases. The intrinsic luminescence almost disappears and is dominated by the green emission band of Mn2+ when the pH increases to 9.5. In this case, the phosphorescence time of Mn2+-doped ZnGa2O4 materials exhibits more than 100 s (Fig. 2b). According to the above results, a possible persistent luminescence mechanism of Mn2+-doped ZnGa2O4 materials is proposed (Fig. 2c)[40, 41]. The excited electrons and holes produced under ultraviolet light excitation are trapped by electron traps and hole traps, respectively[42]. Then, some electrons get away from the electron traps under thermal stimulation and transfer to native defects, thereby resulting in the formation of emission peaks from ZnGa2O4 materials[43]. The other part of the electrons escape and transfer to the excitation level of the Mn2+ ion[44]. Subsequently, the recombination of holes and electrons causes Mn2+ ion to emit green light[45].
Figure 2
2.1.3 Eu2+-doped PLMs
Eu2+ ion is a common doped ion in PLMs. The introduction of Eu2+ ion usually produces yellow, orange and red emissions for the reason that the crystal field reduces the emission energy of 4f65d1 electron configuration of Eu2+ ion[46]. Yang et al. researched the structure and optical performance of Eu2+-doped SrAl2O4 materials[4]. It is found that the crystal phase of the Eu2+-doped SrAl2O4 materials corresponds to the standard pattern, indicating that the structure of the SrAl2O4 materials has not obviously changed after the introduction of Eu2+ ion. Moreover, Eu2+-doped SrAl2O4 shows a strong emission band of 450~700 nm and exhibits a phosphorescence time that exceeds 2 hours. Considering the structure and characteristics of SrAl2O4: Eu2+, a possible persistent luminescence mechanism of SrAl2O4: Eu2+ is deduced. The SrAl2O4 material as the host lattice absorbs the soft X-ray photon energy and stores it in electron traps. Then, the energy gets away from the electron traps and transfers to the 4f65d1 energy level in the Eu2+ ion, which causes the Eu2+ ion to produce radioactive transition luminescence.
2.1.4 B3+-doped PLMs
B3+ ion can replace Ga3+ ion in ZnGa2O4 materials to improve the optical performance of ZnGa2O4 materials because of the similarity of valence and chemical properties between Ga3+ and B3+ ions. Wang et al. researched the changes in the optical performance of ZnGa2O4 materials after B3+-doping[18]. Both ZnGa2O4 and ZnGa2O4: B3+ can detect phosphorescence signal peaks at 502 and 695 nm. However, the phosphorescence decay curves indicate that the phosphorescence intensity and the phosphorescence time of ZnGa2O4: B3+ have been significantly improved compared with ZnGa2O4. According to previous reports, the proper trap depth is essential to achieve a better optical performance of PLMs[1, 47, 48]. There is a bold inference that the introduction of B3+ ion can also increase the content of electron traps
$ {\mathrm{G}\mathrm{a}}_{\mathrm{Z}\mathrm{n}}^{\mathrm{\text{'}}} $ , thus leading to the enhancement of optical performance of ZnGa2O4 after B3+-doping.2.2 Influence of the number of types of doped ions on the structure and optical performance of PLMs
As above mentioned, the structure and optical performance of PLMs are closely related to the type of doped ions. Most PLMs used in practical applications need to be doped with multiple ions to enhance optical performance. The simultaneous introduction of multiple ions will affect not only the host of PLMs, but also the interaction among different ions. Therefore, we introduce the influence of the number of types of doped ions on the structure and optical performance of PLMs in this section.
2.2.1 Ge4+ and Cr3+ co-doped PLMs
The introduction of Ge4+ ion will change the width of the material bandgap due to its unique electronic structure. Thus, the change of structure and optical performance of Ge4+, Cr3+ co-doped PLMs is discussed[5]. Wang et al. found that the crystal phases of the Cr3+-doped ZnGa1.995O4 and Ge4+, Cr3+ co-doped ZnGa1.995O4 materials are corresponding to the standard pattern, indicating that the structure of the materials has not obviously changed after Ge4+, Cr3+ co-doping. In addition, the emission spectra of ZnGa1.995O4: Cr3+ and Zn1.25Ga1.5Ge0.25O4: Cr3+ both present a near-infrared emission band of 650~900 nm[49, 50]. The phosphorescence decay curves show that the optical performance of Zn1.25Ga1.5Ge0.25O4: Cr3+ is better than that of ZnGa1.995O4: Cr3+[5]. Based on the above results, the introduction of Ge4+ improves the filling efficiency of electron traps, thus increasing the phosphorescence time of the materials[23].
2.2.2 Pr3+, Cr3+ co-doped PLMs
The number and depth of the trap energy levels of PLMs are changed after the introduction of Pr3+ ion because of the special 4f5d electronic structure and abundant electronic transition types of Pr3+ ion. Therefore, the changes in structure and optical performance of Zn2.94Ga1.96Ge2O10 after the introduction of Pr3+ and Cr3+ ions are discussed[19]. By analyzing the XRD pattern, the structure of Zn2.94Ga1.96Ge2O10: Cr3+, Pr3+ has not obviously changed, and the pure spinel phase is still maintained. Later, the author does in-depth studies on the optical performance of Zn2.94Ga1.96Ge2O10: Pr3+, Cr3+. The electrons recombine with holes in the natural defects, thereby leading to a wide emission band of 350~660 nm. In addition, the Cr3+ ion provides a near-infrared emission band of 695 nm through the 2E→4A2 transition[27], while the Pr3+ ion increases the phosphorescence time by adjusting the depth and density of traps based on the energy levels of 4f electron configurations[51-55]. Similarly, Yu et al. did further research on the persistent luminescence mechanisms of Zn3Ga2GeO8: Cr3+, Pr3+ to clarify the function of Pr3+ ion[56]. The introduction of Pr3+ ion deepens the trap energy level and increases the number of traps. Given this, a possible persistent luminescence mechanism of Zn3Ga2GeO8: Cr3+, Pr3+ is proposed (Fig. 3). Trap A forms more traps than trap A' by introducing Pr3+ ion, thereby improving the phosphorescence intensity and the phosphorescence time of Zn3Ga2GeO8: Cr3+, Pr3+ materials.
Figure 3
2.2.3 Yb3+, Er3+, Cr3+ co-doped PLMs
Yb3+ ion and Er3+ ion are rare earth element ions used as luminescence centers, which are usually introduced into PLMs to change the density and depth of the trap levels resulting from their unique electronic structure. Consequently, the structure and optical performance of Zn1.25Ga1.5Ge0.25O4: Yb3+, Er3+, Cr3+ (ZGGO: Cr3+, Yb3+, Er3+) with a pure spinel phase is discussed[23]. Yan et al. found that the structure of Zn1.25Ga1.5Ge0.25O4 has not obviously changed after doping with Yb3+, Er3+ and Cr3+ ion. Moreover, ZGGO: Cr3+, Yb3+, Er3 presents an excellent optical performance, and the phosphorescence time exceeds 20 days. Later, the author studied the persistent luminescence mechanism of ZGGO: Yb3+, Er3+, Cr3+ (Fig. 4). The excited electrons are captured by electron traps under ultraviolet light irradiation, and then moves to deep traps by means of nonradiative relaxation. Subsequently, the combination of the excited electrons and the Cr3+ ion leads to the formation of a persistent phosphorescence signal after the ultraviolet radiation is stopped. The introduction of Yb3+ and Er3+ ions can not only provide the materials with additional electrons and energy levels, but also adjust the density and depth of traps[51, 57, 58]. The excited electrons are trapped in the deepest 4f energy levels of Yb3+ and Er3+ ions, thereby extending the time for the trapped electrons to return to the 2E energy level of Cr3+ ion (Fig. 4a, b)[58], which also obviously prolongs the phosphorescence time of materials.
Figure 4
2.2.4 Eu3+, Ti4+ and Mg2+ co-doped PLMs
Viana et al. researched the structure and optical performance of Eu3+, Ti4+, Mg2+ co-doped Gd2O2S materials with a pure hexagonal Gd2O2S phase[20]. The study found that Ti4+ and Mg2+ ions are trapping centers, and Eu3+ ions are the emission centers. The Gd2O2S: Eu3+, Ti4+, Mg2+ has an emission band of 580~720 nm due to the electronic transitions of Eu3+ ion. Therefore, it can be inferred that the Eu3+ ion plays the role of the luminescence center in the materials. Moreover, Gd2O2S: Eu3+, Ti4+, Mg2+ has a longer phosphorescence time compared with Gd2O2S: Eu3+ for the reason that Ti4+ and Mg2+ ions play an important in improving the density of trap energy levels. However, the particle size and crystal structure of Gd2O2S materials have not obviously changed after the introduction of Eu3+, Ti4+ and Mg2+ ions.
2.3 Influence of the content of doped ions on the
2.4 structure and optical performance of PLMs
As mentioned above, some doped ions play the role of the emission center of PLMs, while other doped ions will affect the number and depth of trap levels. Therefore, it is of great necessity to understand how the content of doped ions influences the structure and optical performance of PLMs. This section will discuss how the content of doped ions affects the structure and optical performance of PLMs.
2.3.1 Pr3+, Cr3+ co-doped PLMs
Normally, Cr3+ ion serves as the luminescence center of PLMs, while Pr3+ ion affects the number and depth of trap levels. Yan et al. studied how the contents of Pr3+ and Cr3+ ions influence the structure and optical performance of PLMs. It is found that the increase of Cr3+ content promotes the energy release of Zn2.94Ga1.96Ge2O10: Cr3+, Pr3+. Therefore, as the amount of Cr3+ ion doping increases, the phosphorescence intensity and phosphorescence time of the material decrease[19, 27, 59]. Similarly, Zhang et al. studied the changes in particle size of Pr3+, Cr3+ co-doped Zn2Ga2.98–xGe0.75O8 materials with different Pr3+ contents[22]. It is found that the average size of the materials decreases with the increase of Pr3+ contents. Considering that the doped ions can strongly affect the crystal growth rate through surface charge modification[60], the size reduction of Zn2Ga2.98–xGe0.75O8: Cr3+, Pr3+ may be related to the influence of Pr3+ ion on the surface charge of the material. Specifically, the surface charge distribution of the materials will be changed when the Pr3+ ion is introduced into the materials, thereby reducing the growth rate. Subsequently, the optical performance of Pr3+, Cr3+ co-doped Zn2Ga2.98–xGe0.75O8 materials is researched. As shown in Fig. 5a, the phosphorescence intensity and the phosphorescence time of the materials increase as the increase of Pr3+ doping contents due to the changes in the trap levels of the materials caused by the introduction of Pr3+ ion (Fig. 5b). When the content of Pr3+ ion is too high, the luminescence performance will decrease due to the concentration quenching effect.
Figure 5
Figure 5. (a) Phosphorescence decay curves of Pr3+, Cr3+ co-doped Zn2Ga2.98–xGe0.75O8 materials with different Pr3+ doping contents. The inset presents the phosphorescence intensity of the materials after the ultraviolet light irradiation is stopped, (b) Thermo-luminescence spectra of Pr3+, Cr3+ co-doped Zn2Ga2.98–xGe0.75O8 materials with different Pr3+ doping contents2.3.2 Bi3+, Cr3+ co-doped PLMs
Bi3+ ion can significantly reduce the bandgap energy of PLMs and improve the efficiency of electron trap filling resulting from its unique 6s orbital. Tuerdi et al. researched the influence of crystalline structure, emission peak wavelength and phosphorescence time of ZnGa2O4: Bi3+, Cr3+ materials with different Bi3+ doping contents[16]. The Bi3+ ion tends to replace Ga3+ ion in ZnGa2O4 with a similar ion radius, which makes the material produce higher periodic lattice distortion, thereby making the materials exhibit a longer phosphorescence time. When the doped ratio of Bi3+ ion is low, the crystal phase of Cr3+, Bi3+ co-doped ZnGa2O4 materials corresponds to its standard spectrum, indicating that the structure of ZnGa2O4 materials has not obviously changed after the introduction of Bi3+ ion. However, when the doped ratio of Bi3+ ion increases to 0.03, a strong peak related to the rhombohedral Bi3+ structure at 28.01° is observed in the XRD pattern (Fig. 6a), illustrating that the original crystal structure of the materials is destroyed. Correspondingly, its optical performance is also reduced. The phosphorescence emission spectrum of ZnGa2O4: Bi3+, Cr3+ materials with different Bi3+ doping contents shows a red-shift of emission peak wavelength, which indicates that Bi3+ ion reduces the crystal field intensity of the Cr3+ ion (Fig. 6b).
Figure 6
2.3.3 B3+, Cr3+ co-doped PLMs
As mentioned above, the introduction of B3+ ion can improve the optical performance of ZnGa2O4. On this basis, the researchers studied the influence of doped ion content on PLMs. For example, Yan et al. synthesized ZnGa2O4: B3+, Cr3+ materials by hydrothermal method, and studied the optical performance of ZnGa2O4: Cr3+ with different B3+ doping contents[17]. The XRD pattern presents that the structure of the material has not obviously changed after doping with the B3+ and Cr3+ ions. Besides, the emission wavelength of the materials has not obviously changed when the B3+ ion is introduced into the materials, which indicates that the B3+ ion does not play the role of luminescence center in the materials. At the same time, the improvement of the optical performance of materials shows that the introduction of B3+ ion injects new electron traps into the materials and increases the content of electron traps.
2.3.4 Eu3+, Ti4+ and Mg2+ co-doped PLMs
Eu3+, Ti4+ and Mg2+ ions will act as luminescence centers or trap levels to affect the optical performance of the material. Therefore, studying the optical performance of Gd2O2S: Eu3+, Ti4+ and Mg2+ materials with different doping contents will help us better understand the role of these ions in the material[20]. The study found that Eu3+ ion provides trap levels for electron-hole recombination, and Ti4+ ion provides electron traps to achieve the formation of the phosphorescence signal. Since Ti4+ ion replaces Gd3+ ion and destroys the charge conservation of the materials, it is necessary to introduce Mg2+ ion to maintain charge balance. In addition, as the doping contents of Mg2+ ion increase, the phosphorescence intensity of the material increases. This indicates that the introduction of Mg2+ ion can induce the formation of intermediate trap levels in the material, which prolongs the storage time of the carriers in the material, thus realizing the improvement of the persistent performance of the materials[61].
Apart from the ion-doped PLMs mentioned above, other doped ions also have an impact on the structure and optical performance. For example, Li2ZnGeO4: Mn2+[62], SrAl2O4: Eu2+, Dy3+[63], GdAlO3: Mn4+, Ge4+[64], LaAlO3: Mn4+, Ge4+[65], CaMgSi2O6: Eu2+, Dy3+, Mn2+[66], Y3Al5–xGaxO12: Ce3+, Bi3+ (x = 0~4)[67], MgGeO3: Mn2+, Bi3+[68], Y3Sc2Ga3O12: Ce3+[69], Ca9Bi(PO4)7: Ce3+, Tb3+, Mn2+[70], Ca2BO3Cl: Eu2+, Dy3+[71], Lu2O3: Tb3+, Ca2+[72], KY3F10: Tb3+[73] and AlN: Mn2+[74].
3. CONCLUSION
In this review, we mainly focus on the impact of the type of doped ions, the number of types of doped ions, and the content of doped ions on the structure and optical performance of PLMs. In the past decade, although various doped ions have been widely used to improve the optical performance of PLMs, there is still much work to do in the future. First, in the actual preparation process, the optical performance of PLMs will be affected by the symmetry of matrix lattice, the radius of activated ions, and the electronegativity and distribution of external electron cloud. This should be paid more attention to by researchers. Second, exploring the relationship between doped ions and the host lattice and investigating the influence of the defects caused by doped ions on the storage time of the carriers. At present, these issues still have no exact quantitative relationship, and further researches are needed. Third, understanding the role of trap types, trap contents and probability of trapping electrons in the researches of the persistent luminescence mechanisms. Forth, exploring new doped ions and substrates. For example, most current PLMs are based on aluminates, gallates and silicates matrices. It is necessary to find new matrices with excellent properties. Collectively, with the deepening research of PLMs, ion-doped PLMs with higher luminescence intensity and phosphorescence lifetime will have a broad development prospect.
-
-
[1]
Van den Eeckhout, K.; Smet, P. F.; Poelman, D. Persistent luminescence in Eu2+-doped compounds: a review. Materials 2010, 3, 2536-2566. doi: 10.3390/ma3042536
-
[2]
Zhou, Z.; Zheng, W.; Kong, J.; Liu, Y.; Huang, P.; Zhou, S.; Chen, Z.; Shi, J.; Chen, X. Rechargeable and LED-activated ZnGa2O4: Cr3+ near-infrared persistent luminescence nanoprobes for background-free biodetection. Nanoscale 2017, 9, 6846-6853. doi: 10.1039/C7NR01209H
-
[3]
Song, L.; Li, P. P.; Yang, W.; Lin, X. H.; Liang, H.; Chen, X. F.; Liu, G.; Li, J.; Yang, H. H. Low-dose X-ray activation of W(VI)-doped persistent luminescence nanoparticles for deep-tissue photodynamic therapy. Adv. Funct. Mater. 2018, 28, 1707496-10. doi: 10.1002/adfm.201707496
-
[4]
Song, L.; Lin, X. H.; Song, X. R.; Chen, S.; Chen, X. F.; Li, J.; Yang, H. H. Repeatable deep-tissue activation of persistent luminescent nanoparticles by soft X-ray for high sensitivity long-term in vivo bioimaging. Nanoscale 2017, 9, 2718-2722 doi: 10.1039/C6NR09553D
-
[5]
Liu, Y.; Liu, J. M.; Zhang, D.; Ge, K.; Wang, P.; Liu, H.; Fang, G.; Wang, S. Persistent luminescence nanophosphor involved near-infrared optical bioimaging for investigation of foodborne probiotics biodistribution in vivo: a proof-of-concept study. J. Agric. Food. Chem. 2017, 65, 8229-8240. doi: 10.1021/acs.jafc.7b02870
-
[6]
Xue, Z.; Li, X.; Li, Y.; Jiang, M.; Liu, H.; Zeng, S.; Hao, J. X-ray-activated near-infrared persistent luminescent probe for deep-tissue and renewable in vivo bioimaging. ACS Appl. Mater. Inter. 2017, 9, 22132-22142. doi: 10.1021/acsami.7b03802
-
[7]
Lv, Y.; Ding, D.; Zhuang, Y.; Feng, Y.; Shi, J.; Zhang, H.; Zhou, T. L.; Chen, H.; Xie, R. J. Chromium-doped zinc gallogermanate@zeolitic imidazolate framework-8: a multifunctional nanoplatform for rechargeable in vivo persistent luminescence imaging and pH-responsive drug release. ACS Appl. Mater. Inter. 2019, 11, 1907-1916. doi: 10.1021/acsami.8b19172
-
[8]
Li, Y.; Gecevicius, M.; Qiu, J. Long persistent phosphors-from fundamentals to applications. Chem. Soc. Rev. 2016, 45, 2090-2136. doi: 10.1039/C5CS00582E
-
[9]
Chander, H.; Haranath, D.; Shanker, V.; Sharma, P. Synthesis of nanocrystals of long persisting phosphor by modified combustion technique. J. Cryst. Growth 2004, 271, 307-312. doi: 10.1016/j.jcrysgro.2004.07.026
-
[10]
Lecuyer, T.; Teston, E.; Ramirez Garcia, G.; Maldiney, T.; Viana, B.; Seguin, J.; Mignet, N.; Scherman, D.; Richard, C. Chemically engineered persistent luminescence nanoprobes for bioimaging. Theranostics 2016, 6, 2488-2524. doi: 10.7150/thno.16589
-
[11]
Yamamoto, H.; Matsuzawa, T. Mechanism of long phosphorescence of SrAl2O4: Eu2+, Dy3+ and CaAl2O4: Eu2+, Nd3+. J. Lumin. 1997, 72-74, 287-289. doi: 10.1016/S0022-2313(97)00012-4
-
[12]
Zhuang, Y.; Lv, Y.; Wang, L.; Chen, W.; Zhou, T. L.; Takeda, T.; Hirosaki, N.; Xie, R. J. Trap depth engineering of SrSi2O2N2: Ln2+, Ln3+ (Ln2+ = Yb, Eu; Ln3+ = Dy, Ho, Er) persistent luminescence materials for information storage applications. ACS Appl. Mater. Inter. 2018, 10, 1854-1864. doi: 10.1021/acsami.7b17271
-
[13]
Cui, G.; Yang, X.; Zhang, Y.; Fan, Y.; Chen, P.; Cui, H.; Liu, Y.; Shi, X.; Shang, Q.; Tang, B. Round-the-clock photocatalytic hydrogen production with high efficiency by a long-afterglow material. Angew. Chem. Int. Ed. 2019, 58, 1340-1344. doi: 10.1002/anie.201810544
-
[14]
Liu, J.; Lecuyer, T.; Seguin, J.; Mignet, N.; Scherman, D.; Viana, B.; Richard, C. Imaging and therapeutic applications of persistent luminescence nanomaterials. Adv. Drug Deliver. Rev. 2019, 138, 193-210. doi: 10.1016/j.addr.2018.10.015
-
[15]
Luo, Q.; Wang, W.; Tan, J.; Yuan, Q. Surface modified persistent luminescence probes for biosensing and bioimaging: a review. Chin. J. Chem. 2021, 39, 1009-1021. doi: 10.1002/cjoc.202000583
-
[16]
Tuerdi, A.; Abdukayum, A. Dual-functional persistent luminescent nanoparticles with enhanced persistent luminescence and photocatalytic activity. RSC Adv. 2019, 9, 17653-17657. doi: 10.1039/C9RA02235J
-
[17]
Zhao, H. X.; Yang, C. X.; Yan, X. P. Fabrication and bioconjugation of B(III) and Cr(III) co-doped ZnGa2O4 persistent luminescent nanoparticles for dual-targeted cancer bioimaging. Nanoscale 2016, 8, 18987-18994. doi: 10.1039/C6NR06259H
-
[18]
Li, D.; Wang, Y.; Xu, K.; Zhao, H.; Hu, Z. Effect of H3BO3 on the persistent luminescence and photocatalytic properties of ZnGa2O4 phosphors. Opt. Mater. 2014, 36, 1836-1840. doi: 10.1016/j.optmat.2014.04.027
-
[19]
Abdukayum, A.; Chen, J. T.; Zhao, Q.; Yan, X. P. Functional near infrared-emitting Cr3+/Pr3+ co-doped zinc gallogermanate persistent luminescent nanoparticles with superlong afterglow for in vivo targeted bioimaging. J. Am. Chem. Soc. 2013, 135, 14125-14133. doi: 10.1021/ja404243v
-
[20]
Rosticher, C.; Viana, B.; Fortin, M. A.; Lagueux, J.; Faucher, L.; Chanéac, C. Gadolinium oxysulfide nanoprobes with both persistent luminescent and magnetic properties for multimodal imaging. RSC Adv. 2016, 6, 55472-55478. doi: 10.1039/C6RA05030A
-
[21]
Wang, J.; Ma, Q.; Zheng, W.; Liu, H.; Yin, C.; Wang, F.; Chen, X.; Yuan, Q.; Tan, W. One-dimensional luminous nanorods featuring tunable persistent luminescence for autofluorescence-free biosensing. ACS Nano 2017, 11, 8185-8191. doi: 10.1021/acsnano.7b03128
-
[22]
Gong, Z.; Liu, Y.; Yang, J.; Yan, D.; Zhu, H.; Liu, C.; Xu, C.; Zhang, H. A Pr3+ doping strategy for simultaneously optimizing the size and near infrared persistent luminescence of ZGGO: Cr(3+) nanoparticles for potential bio-imaging. Phys. Chem. Chem. Phys. 2017, 19, 24513-24521. doi: 10.1039/C7CP02909H
-
[23]
Li, Y. J.; Yan, X. P. Synthesis of functionalized triple-doped zinc gallogermanate nanoparticles with superlong near-infrared persistent luminescence for long-term orally administrated bioimaging. Nanoscale 2016, 8, 14965-14970. doi: 10.1039/C6NR04950H
-
[24]
Jiang, R.; Yang, J.; Meng, Y.; Yan, D.; Liu, C.; Xu, C.; Liu, Y. X-ray/red-light excited ZGGO: Cr, Nd nanoprobes for NIR-I/II afterglow imaging. Dalton T. 2020, 49, 6074-6083. doi: 10.1039/D0DT00247J
-
[25]
Wang, J.; Ma, Q.; Wang, Y.; Shen, H.; Yuan, Q. Recent progress in biomedical applications of persistent luminescence nanoparticles. Nanoscale 2017, 9, 6204-6218. doi: 10.1039/C7NR01488K
-
[26]
Singh, S. K. Red and near infrared persistent luminescence nano-probes for bioimaging and targeting applications. RSC Adv. 2014, 4, 58674-58698. doi: 10.1039/C4RA08847F
-
[27]
Bessière, A.; Jacquart, S.; Priolkar, K.; Lecointre, A.; Viana, B.; Gourier, D. ZnGa2O4: Cr3+: a new red long-lasting phosphor with high brightness. Opt. Express 2011, 19, 10131-10137. doi: 10.1364/OE.19.010131
-
[28]
Allix, M.; Chenu, S.; Véron, E.; Poumeyrol, T.; Kouadri Boudjelthia, E. A.; Alahraché, S.; Porcher, F.; Massiot, D.; Fayon, F. Considerable improvement of long-persistent luminescence in germanium and tin substituted ZnGa2O4. Chem. Mater. 2013, 25, 1600-1606. doi: 10.1021/cm304101n
-
[29]
Dhak, P.; Gayen, U. K.; Mishra, S.; Pramanik, P.; Roy, A. Optical emission spectra of chromium doped nanocrystalline zinc gallate. J. Appl. Phys. 2009, 106, 063721-6. doi: 10.1063/1.3224866
-
[30]
Kim, J. S.; Kim, J. S.; Park, H. L. Optical and structural properties of nanosized ZnGa2O4: Cr3+ phosphor. Solid State Commun. 2004, 131, 735-738. doi: 10.1016/j.ssc.2004.07.026
-
[31]
Xu, J.; Murata, D.; Ueda, J.; Viana, B.; Tanabe, S. Toward rechargeable persistent luminescence for the first and third biological windows via persistent energy transfer and electron trap redistribution. Inorg. Chem. 2018, 57, 5194-5203. doi: 10.1021/acs.inorgchem.8b00218
-
[32]
Feng, P.; Li, G.; Guo, H.; Liu, D.; Ye, Q.; Wang, Y. Identifying a cyan ultralong persistent phosphorescence (Ba, Li) (Si, Ge, P)2O5: Eu2+, Pr3+ via solid solution strategy. J. Phys. Chem. C 2019, 123, 3102-3109. doi: 10.1021/acs.jpcc.8b11084
-
[33]
Bai, Q.; Zhao, S.; Guan, L.; Wang, Z.; Li, P.; Xu, Z. Design and control of the luminescence of Cr3+-doped phosphors in the near-infrared I region by fitting the crystal field. Cryst. Growth Des. 2018, 18, 3178-3186. doi: 10.1021/acs.cgd.8b00273
-
[34]
Takahashi, Y.; Ando, M.; Ihara, R.; Fujiwara, T. Green-emissive Mn-activated nanocrystallized glass with willemite-type Zn2GeO4. Opt. Mater. Express 2011, 1, 372-378. doi: 10.1364/OME.1.000372
-
[35]
Terraschke, H.; Wickleder, C. UV, blue, green, yellow, red, and small: newest developments on Eu2+-doped nanophosphors. Chem. Rev. 2015, 115, 11352-11378. doi: 10.1021/acs.chemrev.5b00223
-
[36]
Cheng, J.; Li, P.; Wang, Z.; Li, Z.; Tian, M.; Wang, C.; Yang, Z. Color selective manipulation in Li2ZnGe3O8: Mn2+ by multiple-cation substitution on different crystal-sites. Dalton T. 2018, 47, 4293-4300. doi: 10.1039/C7DT04552B
-
[37]
Li, Z.; Wang, Q.; Wang, Y.; Ma, Q.; Wang, J.; Li, Z.; Li, Y.; Lv, X.; Wei, W.; Chen, L.; Yuan, Q. Background-free latent fingerprint imaging based on nanocrystals with long-lived luminescence and pH-guided recognition. Nano Res. 2018, 11, 6167-6176. doi: 10.1007/s12274-018-2133-6
-
[38]
Feng, Y.; Deng, D.; Zhang, L.; Liu, R.; Lv, Y. LRET-based functional persistent luminescence nanoprobe for imaging and detection of cyanide ion. Sens. Actuators B-Chem. 2019, 279, 189-196. doi: 10.1016/j.snb.2018.09.111
-
[39]
Wang, J.; Ma, Q.; Wang, Y.; Li, Z.; Li, Z.; Yuan, Q. New insights into the structure-performance relationships of mesoporous materials in analytical science. Chem. Soc. Rev. 2018, 47, 8766-8803. doi: 10.1039/C8CS00658J
-
[40]
Liu, H.; Hu, X.; Wang, J.; Liu, M.; Wei, W.; Yuan, Q. Direct low-temperature synthesis of ultralong persistent luminescence nanobelts based on a biphasic solution-chemical reaction. Chin. Chem. Lett. 2018, 29, 1641-1644. doi: 10.1016/j.cclet.2018.02.005
-
[41]
Wang, Y.; Wang, J.; Ma, Q.; Li, Z.; Yuan, Q. Recent progress in background-free latent fingerprint imaging. Nano Res. 2018, 11, 5499-5518. doi: 10.1007/s12274-018-2073-1
-
[42]
Cheng, S.; Shen, B.; Yuan, W.; Zhou, X.; Liu, Q.; Kong, M.; Shi, Y.; Yang, P.; Feng, W.; Li, F. Time-gated ratiometric detection with the same working wavelength to minimize the interferences from photon attenuation for accurate in vivo detection. ACS Cent. Sci. 2019, 5, 299-307. doi: 10.1021/acscentsci.8b00763
-
[43]
Ou, X. Y.; Guo, T.; Song, L.; Liang, H. Y.; Zhang, Q. Z.; Liao, J. Q.; Li, J. Y.; Li, J.; Yang, H. H. Autofluorescence-free immunoassay using X-ray scintillating nanotags. Anal. Chem. 2018, 90, 6992-6997. doi: 10.1021/acs.analchem.8b01315
-
[44]
Wang, J.; Ma, Q.; Liu, H.; Wang, Y.; Shen, H.; Hu, X.; Ma, C.; Yuan, Q.; Tan, W. Time-gated imaging of latent fingerprints and specific visualization of protein secretions via molecular recognition. Anal. Chem. 2017, 89, 12764-12770. doi: 10.1021/acs.analchem.7b03003
-
[45]
Shen, H.; Wang, Y.; Wang, J.; Li, Z.; Yuan, Q. Emerging biomimetic applications of DNA nanotechnology. ACS Appl. Mater. Inter. 2019, 11, 13859-13873. doi: 10.1021/acsami.8b06175
-
[46]
Jia, D.; Jia, W.; Evans, D. R.; Dennis, W. M.; Liu, H.; Zhu, J.; Yen, W. M. Trapping processes in CaS: Eu2+, Tm3+. J. Appl. Phys. 2000, 88, 3402-3407. doi: 10.1063/1.1286419
-
[47]
Guo, C.; Tang, Q.; Huang, D.; Zhang, C.; Su, Q. Tunable color emission and afterglow in CaGa2S4: Eu2+, Ho3+ phosphor. Mater. Res. Bull. 2007, 42, 2032-2039. doi: 10.1016/j.materresbull.2007.02.023
-
[48]
Denis, G.; Deniard, P.; Rocquefelte, X.; Benabdesselam, M.; Jobic, S. The thermally connected traps model applied to the thermoluminescence of Eu2+ doped Ba13-xAl22-2xSi10+2xO66 materials (x~0.6). Opt. Mater. 2010, 32, 941-945. doi: 10.1016/j.optmat.2010.01.029
-
[49]
Struve, B.; Huber, G. The effect of the crystal field strength on the optical spectra of Cr3+ in gallium garnet laser crystals. Appl. Phys. B 1985, 36, 195-201. doi: 10.1007/BF00704574
-
[50]
Forster, L. S. The photophysics of chromium(III) complexes. Chem. Rev. 1990, 90, 331-353. doi: 10.1021/cr00100a001
-
[51]
Maldiney, T.; Lecointre, A.; Viana, B.; Bessiere, A.; Bessodes, M.; Gourier, D.; Richard, C.; Scherman, D. Controlling electron trap depth to enhance optical properties of persistent luminescence nanoparticles for in vivo imaging. J. Am. Chem. Soc. 2011, 133, 11810-11815. doi: 10.1021/ja204504w
-
[52]
Jia, G.; Lewis, L.; Wang, X. Cr(3+)-doped lanthanum gallogermanate phosphors with long persistent IR emission. Electrochem. Solid St. 2010, 13, J32-J34. doi: 10.1149/1.3294520
-
[53]
Aitasalo, T.; Dereń, P.; Hölsä, J.; Jungner, H.; Krupa, J. C.; Lastusaari, M.; Legendziewicz, J.; Niittykoski, J.; Stręk, W. Persistent luminescence phenomena in materials doped with rare earth ions. J. Solid State Chem. 2003, 171, 114-122. doi: 10.1016/S0022-4596(02)00194-9
-
[54]
Li, X.; Zhang, F.; Zhao, D. Highly efficient lanthanide upconverting nanomaterials: progresses and challenges. Nano Today 2013, 8, 643-676. doi: 10.1016/j.nantod.2013.11.003
-
[55]
Dong, H.; Sun, L. D.; Yan, C. H. Basic understanding of the lanthanide related upconversion emissions. Nanoscale 2013, 5, 5703-5714. doi: 10.1039/c3nr34069d
-
[56]
Cai, Y.; Liu, B.; Chen, W.; Qiu, J.; Xu, X.; Zhao, L.; Yu, X. X-ray and UV excited long persistent luminescence properties of Zn3Ga2GeO8: Cr3+, Pr3+. ECS J. Solid State Sc. 2020, 9, 066006-7. doi: 10.1149/2162-8777/aba852
-
[57]
Sun, S. K.; Wang, H. F.; Yan, X. P. Engineering persistent luminescence nanoparticles for biological applications: from biosensing/bioimaging to theranostics. Acc. Chem. Res. 2018, 51, 1131-1143. doi: 10.1021/acs.accounts.7b00619
-
[58]
Qu, B.; Zhang, B.; Wang, L.; Zhou, R.; Zeng, X. C. Mechanistic study of the persistent luminescence of CaAl2O4: Eu, Nd. Chem. Mater. 2015, 27, 2195-2202. doi: 10.1021/acs.chemmater.5b00288
-
[59]
Pan, Z.; Lu, Y. Y.; Liu, F. Sunlight-activated long-persistent luminescence in the near-infrared from Cr3+-doped zinc gallogermanates. Nat. Mater. 2011, 11, 58-63.
-
[60]
Wang, F.; Han, Y.; Lim, C. S.; Lu, Y.; Wang, J.; Xu, J.; Chen, H.; Zhang, C.; Hong, M.; Liu, X. Simultaneous phase and size control of upconversion nanocrystals through lanthanide doping. Nature 2010, 463, 1061-1065. doi: 10.1038/nature08777
-
[61]
Mikami, M.; Oshiyama, A. First-principles study of intrinsic defects in yttrium oxysulfide. Phys. Rev. B 1999, 60, 1707-1715. doi: 10.1103/PhysRevB.60.1707
-
[62]
Li, P.; Peng, M.; Wondraczek, L.; Zhao, Y.; Viana, B. Red to near infrared ultralong lasting luminescence from Mn2+-doped sodium gallium aluminum germanate glasses and (Al, Ga)-albite glass-ceramics. J. Mater. Chem. C 2015, 3, 3406-3415. doi: 10.1039/C5TC00047E
-
[63]
Kandpal, S. K.; Goundie, B.; Wright, J.; Pollock, R. A.; Mason, M. D.; Meulenberg, R. W. Investigation of the emission mechanism in milled SrAl2O4: Eu, Dy using optical and synchrotron X-ray spectroscopy. ACS Appl. Mater. Inter. 2011, 3, 3482-3486. doi: 10.1021/am200710j
-
[64]
Liu, J. M.; Liu, Y. Y.; Zhang, D. D.; Fang, G. Z.; Wang, S. Synthesis of GdAlO3: Mn4+, Ge4+@Au core-shell nanoprobes with plasmon-enhanced near-infrared persistent luminescence for in vivo trimodality bioimaging. ACS Appl. Mater. Inter. 2016, 8, 29939-29949. doi: 10.1021/acsami.6b09580
-
[65]
Li, Y.; Li, Y. Y.; Sharafudeen, K.; Dong, G. P.; Zhou, S. F.; Ma, Z. J.; Peng, M. Y.; Qiu, J. R. A strategy for developing near infrared long-persistent phosphors: taking MAlO3: Mn4+, Ge4+ (M = La, Gd) as an example. J. Mater. Chem. C 2014, 2, 2019-2027. doi: 10.1039/c3tc32075h
-
[66]
Rosticher, C.; Viana, B.; Laurent, G.; Le Griel, P.; Chanéac, C. Insight into CaMgSi2O6: Eu2+, Mn2+, Dy3+ nanoprobes: influence of chemical composition and crystallinity on persistent red luminescence. Eur. J. Inorg. Chem. 2015, 3681-3687.
-
[67]
Katayama, Y.; Hashimoto, A.; Xu, J.; Ueda, J.; Tanabe, S. Thermoluminescence investigation on Y3Al5-xGaxO12: Ce3+-Bi3+ green persistent phosphors. J. Lumin. 2017, 183, 355-359. doi: 10.1016/j.jlumin.2016.11.074
-
[68]
Zhuang, Y.; Katayama, Y.; Ueda, J.; Tanabe, S. A brief review on red to near-infrared persistent luminescence in transition-metal-activated phosphors. Opt. Mater. 2014, 36, 1907-1912. doi: 10.1016/j.optmat.2014.05.035
-
[69]
Ueda, J.; Aishima, K.; Nishiura, S.; Tanabe, S. Afterglow luminescence in Ce3+-doped Y3Sc2Ga3O12 ceramics. Appl. Phys. Express 2011, 4, 042602-3. doi: 10.1143/APEX.4.042602
-
[70]
Li, K.; Shang, M.; Zhang, Y.; Fan, J.; Lian, H.; Lin, J. Photoluminescence properties of single-component white-emitting Ca9Bi(PO4)7: Ce3+, Tb3+, Mn2+ phosphors for UV LEDs. J. Mater. Chem. C 2015, 3, 7096-7104. doi: 10.1039/C5TC00927H
-
[71]
Zeng, W.; Wang, Y.; Han, S.; Chen, W.; Li, G.; Wang, Y.; Wen, Y. Design, synthesis and characterization of a novel yellow long-persistent phosphor: Ca2BO3Cl: Eu2+, Dy3+. J. Mater. Chem. C 2013, 1, 3004-3011. doi: 10.1039/c3tc30182f
-
[72]
Trojan Piegza, J.; Niittykoski, J.; Hölsä, J.; Zych, E. Thermoluminescence and kinetics of persistent luminescence of vacuum-sintered Tb3+-doped and Tb3+, Ca2+-codoped Lu2O3 materials. Chem. Mater. 2008, 20, 2252-2261. doi: 10.1021/cm703060c
-
[73]
Cao, C.; Guo, S.; Moon, B. K.; Choi, B. C.; Jeong, J. H. Synthesis, grouping, and optical properties of REF3-KF nanocrystals. Mater. Chem. Phys. 2013, 139, 609-615. doi: 10.1016/j.matchemphys.2013.02.005
-
[74]
Zhang, H.; Zheng, M.; Lei, B.; Liu, Y.; Xiao, Y.; Dong, H.; Zhang, Y.; Ye, S. Luminescence properties of red long-lasting phosphorescence phosphor AlN: Mn2+. ECS J. Solid State Sci. Technol. 2013, 2, R117-R120. doi: 10.1149/2.006307jss
-
[1]
-
Figure 5 (a) Phosphorescence decay curves of Pr3+, Cr3+ co-doped Zn2Ga2.98–xGe0.75O8 materials with different Pr3+ doping contents. The inset presents the phosphorescence intensity of the materials after the ultraviolet light irradiation is stopped, (b) Thermo-luminescence spectra of Pr3+, Cr3+ co-doped Zn2Ga2.98–xGe0.75O8 materials with different Pr3+ doping contents
Table 1. Details of Host, Emitter, Co-dopants, Emission Region and Afterglow Decay of PLMs
Host Emitter Co-dopants Emission region Afterglow decay Ref. SrAl2O4 Eu2+ 450~700 nm > 2 h 4 GdAlO3 Mn2+ Ge4+ 600~840 nm > 1200 s 64 Y3Al5–xGaxO12 (x = 0~4) Ce3+ Bi3+ 505 nm 54~68 min 67 ZnGa2O4 B3+ 502, 695 nm 600 s 18 Zn1.25Ga1.5Ge0.25O4 Cr3+ Yb3+, Er3+ 650~850 nm > 480 h 23 Li2ZnGeO4 Mn2+ 550~800 nm > 960 h 62 MgGeO3 Mn2+ Bi3+ 680 nm 100 min 68 Y3Sc2Ga3O12 Ce3+ 500 nm 100 min 69 CaMgSi2O6 Eu2+ Dy3+, Mn2+ 468, 600, 670 nm > 600s 66 Ca9Bi(PO4)7 Ce3+ Tb3+, Mn2+ 300~500 nm 5 h 70 Ca2BO3Cl Eu2+ Dy3+ 580 nm 48 h 71 Gd2O2S Eu3+ Ti4+, Mg2+ 580~720 nm 120 s 20 Lu2O3 Tb3+ Ca2+ 543 nm 20~30 h 72 KY3F10 Tb3+ 542 nm 108 s 73 AlN Mn2+ 570~700 nm 1 h 74 -

计量
- PDF下载量: 3
- 文章访问数: 334
- HTML全文浏览量: 25